Understanding Electric Semiconductors: Principles and Applications
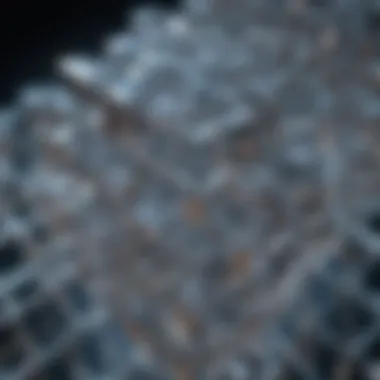
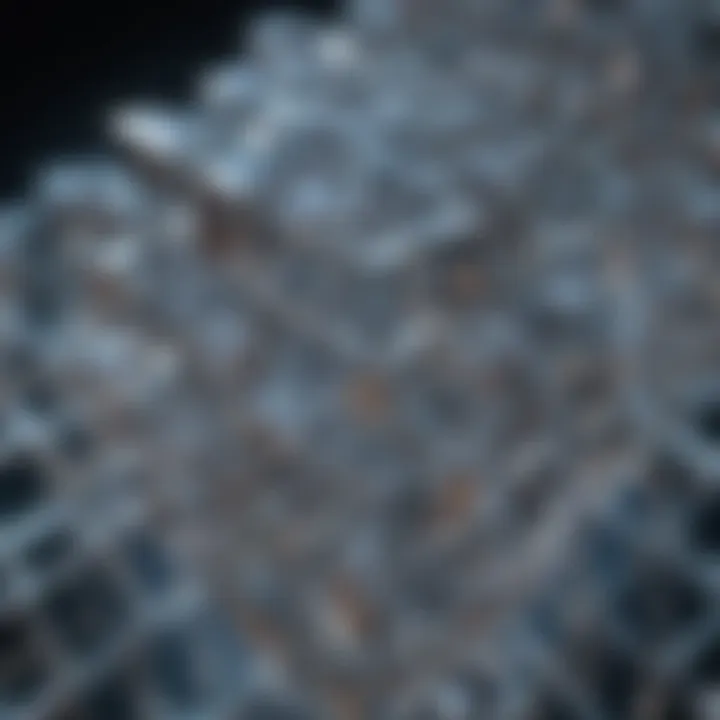
Intro
Electric semiconductors are foundational to modern technology, underpinning a wide array of devices we use every day. They are materials whose electrical properties sit between those of conductors and insulators, making them essential for controlling electrical currents. This article seeks to unpack the complex world of semiconductors, exploring both their intrinsic characteristics and the practical implications of their use across various industries.
Understanding the principles behind semiconductors, such as band theory and electron mobility, provides clarity on their operation in transistors, diodes, and integrated circuits. Furthermore, this exploration takes into account recent advancements in technology and manufacturing processes, which contribute to the growing relevance of semiconductors in renewable energy, computing, and telecommunications.
As we delve deeper into this realm, the significance of electric semiconductors will become evident. They not only facilitate the functioning of everyday gadgets but also drive innovation in sectors like automotive, healthcare, and information technology. The following sections will systematically break down this intricate subject to ensure a comprehensive grasp of both theoretical principles and applied technology.
Preamble to Electric Semiconductors
Electric semiconductors are critical to many technologies we rely on daily. They form the backbone of a range of devices, from simple electronics to complex computing systems. Understanding how these materials work can unlock insights into the future of innovation and technology.
Definition and Characteristics
Electric semiconductors are materials that have an electrical conductivity between that of a conductor and an insulator. This unique property allows them to control the flow of electric current effectively. The primary characteristic of semiconductors is their band structure, particularly the band gap, which determines their conductivity at varying temperatures. Common semiconductors include silicon and gallium arsenide. These materials can be further categorized into intrinsic and extrinsic types, where impurities are added to enhance their properties.
Beyond electrical conductivity, semiconductors possess other important characteristics. They can exhibit photovoltaic effects, allowing them to convert light into electricity, which is crucial for solar energy applications. Moreover, many semiconductors demonstrate non-linear behavior, making them essential in applications like diodes and transistors.
Historical Context and Development
The development of electric semiconductors has a rich history intertwined with technological evolution. The concept of semiconductivity was first recognized in the late 19th century. Early studies involved materials such as selenium and galena, which showed some conductive properties under certain conditions.
The breakthrough came in the 1940s with the invention of the transistor at Bell Labs. John Bardeen, Walter Brattain, and William Shockley developed this device, marking a significant shift in electronic engineering. The transistor's ability to amplify signals played a critical role in the miniaturization of electronics and paved the way for the first integrated circuits in the 1960s.
As technology advanced, so did the methods of manufacturing and doping semiconductors. Innovations in crystal growth and deposition techniques led to the development of more efficient semiconductors. Today, the semiconductor industry is on the cutting edge of innovation, with ongoing research focusing on novel materials and applications.
Thus, the historical development of electric semiconductors not only reflects technological advances but also demonstrates the interplay between science and industry in driving forward our electronic capabilities.
Fundamental Principles of Semiconductors
Semiconductors form the backbone of modern electronic devices. Their unique properties allow them to conduct electricity under certain conditions while acting as insulators under others. This fundamental behavior is pivotal for various applications, making the study of semiconductors essential for anyone involved in electronic technology. Understanding these principles lays the groundwork for innovations across multiple sectors.
Band Theory and Energy Levels
At the heart of semiconductor physics lies band theory. It describes the energy levels available to electrons within a material. In simple terms, these energy levels are divided into two primary bands: the valence band and the conduction band.
The valence band is filled with electrons, while the conduction band is typically empty in a pure semiconductor. The energy gap between these two bands is known as the bandgap. The bandgap is crucial because it determines a semiconductor's electrical properties. For example:
- Small bandgap materials (like germanium) can conduct electricity at lower energies.
- Larger bandgap materials (like silicon) require more energy for electrons to jump into the conduction band.
Understanding band theory is fundamental in predicting how a semiconductor behaves when exposed to external energy sources, such as heat or light. Advanced applications, such as photonics or thermoelectrics, leverage this knowledge to create functional devices.
Intrinsic vs. Extrinsic Semiconductors
Semiconductors can be classified into two categories: intrinsic and extrinsic.
- Intrinsic semiconductors are pure materials without any significant impurities. Their electrical properties are inherently determined by their atomic structure. The behavior of intrinsic semiconductors is essentially based on thermal energy, which encourages some electrons to migrate from the valence band to the conduction band.
- Extrinsic semiconductors are those that have been intentionally doped with impurities to enhance their electrical characteristics. Doping introduces additional energy levels into the band structure, which can significantly improve conductivity. There are two types of extrinsic semiconductors:
- N-type: Doping introduces extra electrons into the conduction band, increasing negative charge carriers.
- P-type: Doping creates holes in the valence band, which act as positive charge carriers.
Understanding the difference between these types is crucial for designing and utilizing semiconductor devices effectively, especially in advanced technologies.
Carrier Concentration and Mobility
Carrier concentration refers to the number of charge carriers (electrons and holes) in a semiconductor material. This factor influences the conductivity of the material. A higher carrier concentration leads to better electrical conduction.
Mobility, on the other hand, defines how quickly these carriers can move through the semiconductor when an electric field is applied. The relationship between carrier concentration and mobility is often represented by the equation:
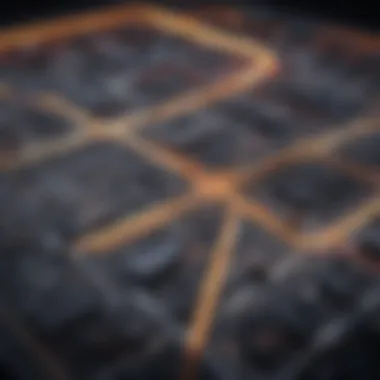
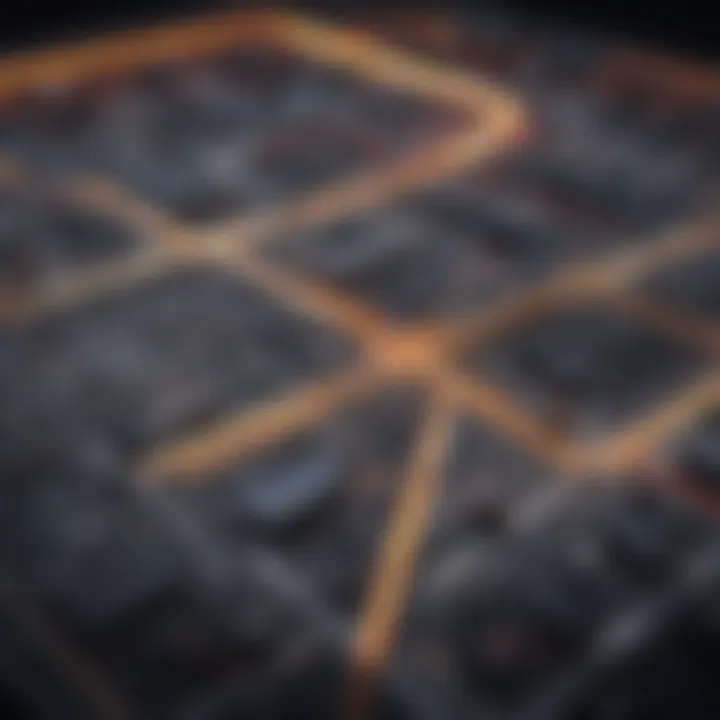
σ = q(nμ_n + pμ_p)
Where:
σ = conductivity
q = charge of an electron
n = electron concentration
μ_n = electron mobility
p = hole concentration
μ_p = hole mobility
These parameters are critical for predicting the performance of semiconductor devices under various conditions. Enhancing both carrier concentration and mobility can lead to better device performance, something that researchers continuously strive for.
By grasping these fundamental principles, engineers and scientists can innovate and optimize semiconductor technologies for future applications.
Types of Semiconductor Materials
Understanding the various types of semiconductor materials is critical in comprehending how electric semiconductors function in different applications. Semiconductor materials are fundamental for the operation of devices like diodes, transistors, and integrated circuits. Each material brings unique properties that influence performance in electronic devices.
Silicon Semiconductors
Silicon is the most widely used semiconductor material due to its abundance and favorable electrical properties. This element exhibits a diamond cubic crystal structure, making it highly stable. Silicon’s band gap is around 1.1 eV, allowing it to operate efficiently at room temperature while still being effective under various conditions.
The process of creating silicon semiconductors often involves techniques such as doping, where impurities are added to enhance conductivity. This method allows silicon to form p-type and n-type semiconductors, which are essential for constructing p-n junctions, a vital component in many electronic devices.
Silicon's reliability and cost-effectiveness make it the backbone of modern electronics.
Gallium Arsenide Semiconductors
Gallium arsenide semiconductors are another pivotal type, particularly valued for their high electron mobility. With a direct band gap of approximately 1.4 eV, gallium arsenide can emit light efficiently, making it a preferred choice for optoelectronic devices, including lasers and LEDs.
The thermal conductivity of gallium arsenide is lower than that of silicon, which can limit its effectiveness in high-temperature applications. However, it is still widely used in high-frequency applications because of its superior performance in microwave and radiofrequency technologies. Its applications often extend to military communication systems and satellite technologies.
Organic Semiconductors
Organic semiconductors differ significantly from inorganic types like silicon or gallium arsenide. Composed of carbon-based materials, they exhibit unique properties, including flexibility, lightweight nature, and compatibility with large-area and low-cost manufacturing techniques.
Organic semiconductors are commonly used in organic light-emitting diodes (OLEDs) and organic photovoltaic cells. These materials support innovative applications, enabling devices like flexible displays and lightweight solar panels. However, their limitations include lower charge carrier mobility and stability in comparison to traditional semiconductors.
Overall, each type of semiconductor material has specific advantages and drawbacks, making them suitable for different applications in technology. Understanding these variations is key for advancing semiconductor designs and innovations.
Manufacturing Processes
Manufacturing processes are crucial in the semiconductor industry since they affect the performance and reliability of semiconductor devices. Each step in these processes shapes the final product, influencing material properties and electronic characteristics. Understanding these processes provides insight into how high-quality semiconductors are produced. This section will explore several methods that are fundamental in creating semiconductors, namely crystal growth techniques, doping methods, and thin film deposition techniques.
Crystal Growth Techniques
Crystal growth techniques play a significant role in fabricating semiconductors. The quality of the semiconductor material depends largely on the method used for crystal growth. Good quality crystals have a tightly packed atomic structure, essential for effective electrical performance.
Several methods exist for crystal growth, with the Czochralski method and the Bridgman method being among the most popular. In the Czochralski method, a seed crystal is dipped into molten semiconductor material, which is then slowly pulled upward while rotating. This results in a single crystal ingot. Conversely, the Bridgman method involves slowly cooling a molten semiconductor within a crucible, allowing for the solidification of a crystal structure. Both methods have specific applications, but they require precise control of temperature and environmental conditions to achieve optimal results.
High-quality semiconductor crystals lead to enhanced performance in electronic devices, reducing the likelihood of defects and improving yield rates during manufacturing.
Doping Methods
Doping is the process of intentionally introducing impurities into a semiconductor to modify its electrical properties. The type of dopant chosen plays a critical role in determining whether the material will be n-type or p-type. N-type semiconductors have excess electrons, while p-type semiconductors have holes, or positive charge carriers.
Common doping methods include ion implantation and diffusion. In ion implantation, ions of the dopant are accelerated and implanted into the semiconductor at specific depths. This method offers precise control over dopant concentration and distribution. On the other hand, diffusion involves heating the semiconductor in the presence of a dopant material, allowing the dopant atoms to diffuse into the crystal lattice. Distinct benefits exist for each method, but ion implantation widely prevails in modern semiconductor manufacturing due to its accuracy.
Thin Film Deposition Techniques
Thin film deposition techniques are valuable for fabricating semiconductor devices with layers of materials. Thin films allow for the development of multi-layer devices, crucial for integrated circuits and solar cells. Different techniques yield discrete advantages depending on the desired properties of the thin film.
Chemical vapor deposition and physical vapor deposition are the two main categories. In chemical vapor deposition (CVD), gaseous reactants form a solid film on the substrate, often utilized in producing high-quality films with conformal coverage. Physical vapor deposition (PVD), including sputtering and evaporation, involves the physical transfer of materials from a target to a substrate. Each of these methods has its trade-offs, with CVD generally favoring uniform films while PVD can achieve high deposition rates with flexible materials.
Applications of Electric Semiconductors
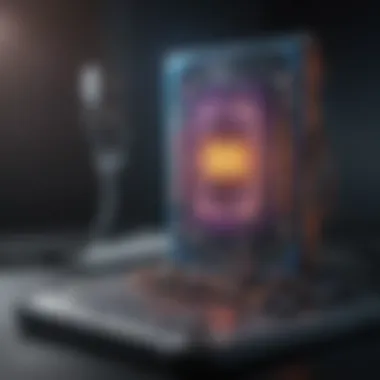
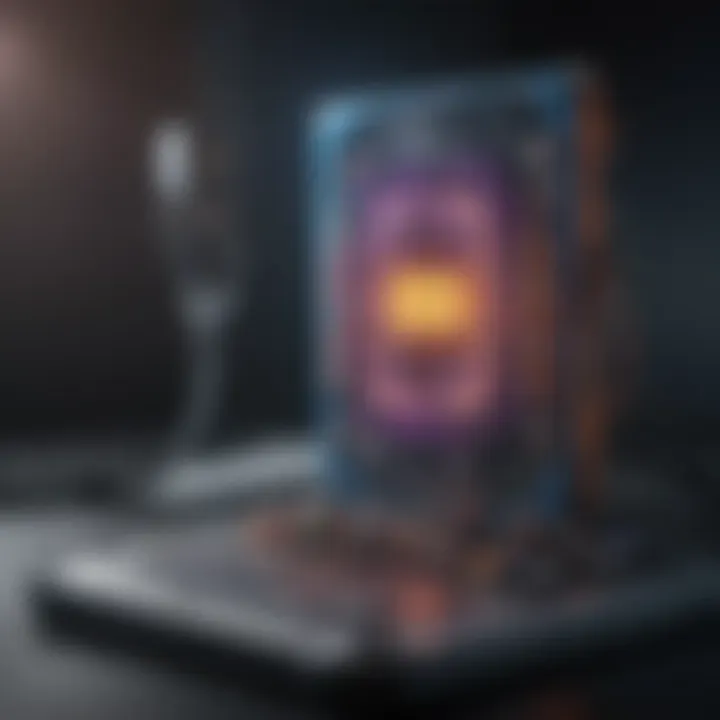
Electric semiconductors play a crucial role across various domains in technology. Their unique properties enable them to function effectively as switches, amplifiers, and signal modulators. Electric semiconductors facilitate the miniaturization of devices, contributing to the creation of compact and highly efficient electronic systems.
Electronics and Consumer Devices
The significance of semiconductors in electronics is immense. They are essential in devices such as smartphones, tablets, and laptops that dominate daily life. Silicon-based semiconductors, for instance, are central to the operation of integrated circuits, enabling the processing and storage of data. These materials allow for the rapid switching of electrical currents, which is necessary for computation and communication.
In consumer devices, semiconductors are responsible for features such as touch screens and energy-efficient LED displays. Moreover, the rise of the Internet of Things (IoT) highlights the increasing demand for semiconductor technologies that can support smart devices. With their role in sensor integration and wireless communication, semiconductors augment the functionalities of modern appliances, creating a seamless user experience.
Renewable Energy Solutions
Electric semiconductors are vital for renewable energy technologies. Photovoltaic cells, made from semiconductor materials like silicon, convert sunlight into electricity efficiently. This capability is crucial for increasing the adoption of solar power, promoting sustainability and reducing reliance on fossil fuels. Additionally, semiconductors are essential in power inverters, which convert direct current (DC) from solar panels into alternating current (AC) usable by the electrical grid.
Energy storage systems also employ semiconductors in their management and conversion processes. By enhancing system efficiencies, they allow for better integration of renewable sources into existing energy frameworks. As the world shifts towards greener energies, the demand for advanced semiconductor applications in this sector will likely increase.
Telecommunications Systems
In telecommunications, electric semiconductors are foundational. They are used in devices that facilitate communication, such as routers, switches, and signal boosters. Semiconductor technologies enable high-speed internet connections and efficient data transmission, thus supporting global communication infrastructures.
Moreover, advancements in semiconductor design improve the speed and capacity of networks. Fiber optics systems, leveraging semiconductor lasers, increase data transmission rates exponentially. As 5G technology emerges, semiconductors will continue to enhance connectivity, enabling innovative applications like remote surgery and autonomous vehicles.
"The influence of electric semiconductors permeates virtually every aspect of modern technology, underlining their indispensable role in our daily lives."
In summary, electric semiconductors are integral not only to consumer electronics but also to renewable energy and telecommunications sectors. Their applications are diverse and continually evolving, fostering innovations that impact society positively. Understanding these applications provides insight into the critical nature of semiconductor technologies in our modern world.
Innovations in Semiconductor Technology
Innovations in semiconductor technology are pivotal for the advancement of various industries, greatly impacting electronics, telecommunications, and renewable energy sectors. These innovations push the boundaries of what is possible with electronic devices, facilitating performance improvements, increased efficiency, and the introduction of entirely new functionalities. As we delve into this realm, we will explore specific elements like advancements in nanotechnology, development of quantum dots, and the emergence of 2D materials.
Advancements in Nanotechnology
Nanotechnology has become a game changer in the semiconductor field. By operating at the nanoscale, researchers can manipulate materials with unmatched precision. This level of control opens doors to creating smaller, more efficient devices. For example, the ability to engineer materials at the molecular level allows for better performance and heat management in electronic circuits.
One significant benefit of using nanotechnology is the enhancement of carrier mobility. This attribute is crucial for the performance of transistors and diodes. Higher mobility leads to faster operation speeds, which is essential for high-frequency applications.
Consider the development of FinFET technology, which utilizes a three-dimensional structure to improve performance. These transistors are not only smaller but offer lower power consumption. Such advancements point toward a future where devices are both compact and powerful, suitable for the increasing demand for mobile computing and data processing.
Development of Quantum Dots
Quantum dots represent a transformative shift in the design of semiconductor materials. These tiny semiconductor particles can emit light of various colors, depending on their size. This tunable property makes them especially useful in display technologies and solar cells. By adjusting the size of the quantum dots, manufacturers can fine-tune the emission spectrum, enhancing color quality and energy efficiency.
Additionally, quantum dots facilitate advancements in quantum computing. Their unique electronic properties enable the creation of qubits, the fundamental units of quantum information. This innovation has the potential to revolutionize computing power by performing tasks that standard computers cannot accomplish within a reasonable timeframe.
Medical applications of quantum dots are emerging. They can be used in imaging and diagnostics, allowing for precise targeting of cancer cells. The combination of semiconductor technology with biological applications represents a significant interdisciplinary leap.
Emergence of 2D Materials
The introduction of 2D materials, such as graphene and transition metal dichalcogenides, has expanded the landscape of semiconductor technology. These materials possess remarkable electrical, mechanical, and thermal properties. For instance, graphene is known for its exceptional conductivity and strength, making it an ideal candidate for many applications.
2D materials allow for thinner and more efficient electronic components. Their unique structure contributes to reduced power loss and enhanced performance. As a result, they are being explored for various applications, including flexible electronics and advanced sensors.
Furthermore, the research on integrating 2D materials with traditional silicon-based technologies is ongoing. This integration promises to enhance device performance while maintaining compatibility with existing manufacturing processes, thereby smoothing the transition to new technologies.
"Innovations in semiconductor technology drive not only performance but also redefine possible applications across various sectors, including healthcare, energy, and computing."
In summary, the innovations in semiconductor technology herald a new era of electronics. The advancing fields of nanotechnology, quantum dots, and 2D materials are redefining industry standards, leading to devices that are smaller, faster, and more efficient. As research continues, we can expect even more groundbreaking developments that will play a crucial role in technological progress.
Challenges Faced by the Semiconductor Industry
The semiconductor industry is pivotal in driving technological advancement across various sectors. However, several challenges confront its growth and sustainability. These obstacles merit thorough examination as they impact supply, innovation, and environmental practices. Understanding these challenges helps us identify potential solutions and future trends in semiconductor technology, emphasizing its importance for both academics and industry professionals.
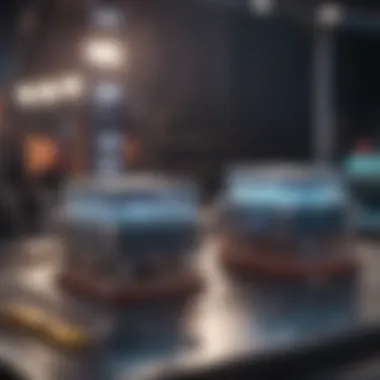
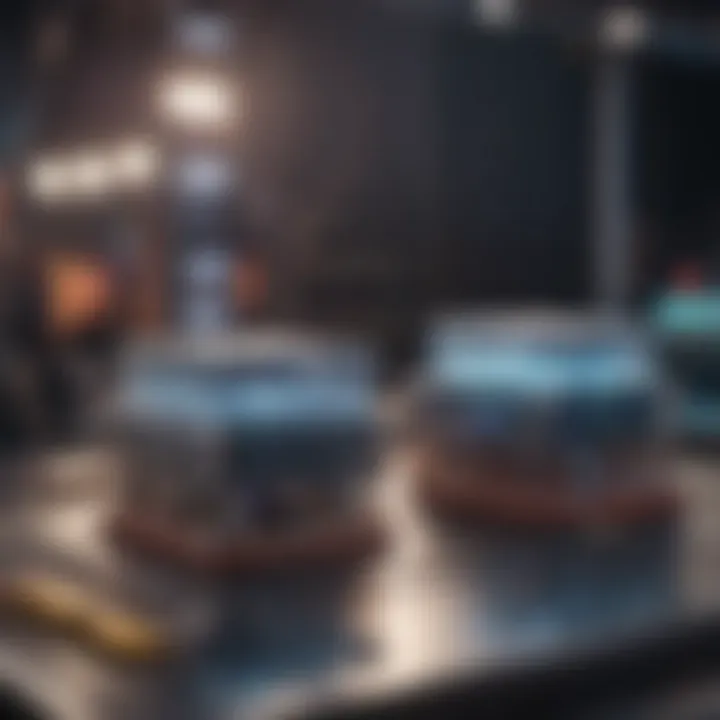
Material Limitations
Material limitations refer to the inherent properties of semiconductor materials, which can restrict their performance and applications. Semiconductor materials like silicon and gallium arsenide have specific advantages but also limitations in terms of efficiency, thermal stability, and scaling potential.
For instance, silicon is widely used due to its cost-effectiveness and good electronic properties. Nevertheless, it faces challenges, such as low electron mobility, which can hinder high-frequency applications. Researchers are exploring alternatives like gallium nitride, which offers higher efficiency and breakdown voltage but comes with higher production costs.
The development of new materials, such as organic semiconductors and 2D materials, shows promise. However, these materials often lack the established manufacturing processes or may run into difficulties regarding scalability and integration with existing technologies.
Supply Chain Issues
The global semiconductor supply chain has become increasingly complex and vulnerable. Disruptions can occur at multiple points, from raw material sourcing to manufacturing and distribution. Events such as the COVID-19 pandemic have highlighted these vulnerabilities, causing significant delays and shortages that impacted multiple industries, including automotive and consumer electronics.
Several factors contribute to supply chain challenges:
- Geopolitical tensions: Trade policies and tariffs between countries can influence material availability.
- Concentration of production: A significant portion of semiconductor manufacturing occurs in specific regions, making the industry susceptible to localized disruptions.
- Inventory management: Just-in-time manufacturing practices can exacerbate shortages during unforeseen events.
These issues often require companies to rethink their supply chains for greater resilience and flexibility, which in turn can lead to higher costs.
Environmental Concerns
The semiconductor industry has been criticized for its environmental footprint during manufacturing. The production process consumes substantial amounts of energy and water while generating hazardous waste. As a result, there is growing pressure from regulatory bodies and the public to adopt sustainable practices.
Key environmental concerns include:
- Energy consumption: Semiconductor fabs require considerable energy, often sourced from non-renewable activities, contributing to carbon emissions.
- Water usage: Water is a critical resource in fabrication processes, leading to concerns over depletion and waste management.
- Chemical waste: The use of toxic chemicals in semiconductor manufacturing poses risks to the environment and human health if not managed properly.
Efforts are being made to mitigate these issues, including developing more energy-efficient manufacturing techniques, recycling processes, and utilizing renewable energy sources. Such practices are not only crucial for compliance but also for long-term sustainability in the industry.
The semiconductor industry must confront its challenges to maintain its role as a cornerstone of modern technology. Recognizing these pressures is vital for driving innovation and sustainable growth within the field.
Future Outlook of Electric Semiconductors
The future of electric semiconductors is poised to be transformative in various sectors. As technology evolves, so does the need for improved semiconductor materials and methods. This section will elaborate on the essential advancements shaping their trajectory, such as research breakthroughs and the role of artificial intelligence. These elements are vital in predicting how semiconductors will support upcoming innovations and challenges in the industry.
Trends in Research and Development
Research in semiconductors is increasingly centered on maximising efficiency and performance. The following trends showcase how the industry is evolving:
- Flexible and Wearable Devices: Researchers are developing new materials that can be integrated into flexible electronics. These innovations will enhance the comfort and user experience in wearable technology.
- Quantum Computing: The drive towards quantum processors requires semiconductors with exceptional purity and precise control of quantum states. Efforts in refining material properties are crucial.
- 3D Semiconductor Structures: This technology allows for denser packing of components, leading to improved performance in smaller packages. It is significant for mobile devices and high-performance computing.
- Energy Efficiency: With growing concerns about sustainability, there is a focus on improving the energy efficiency of semiconductor devices. This includes research into low-energy consumption and eco-friendly materials.
These trends signal an exciting future, where the integration of advanced features in devices will yield enhanced functionalities across various applications.
The Role of Artificial Intelligence
Artificial intelligence (AI) is becoming increasingly influential in the semiconductor industry. Its role can be dissected into various impactful areas:
- Design Optimization: AI algorithms optimize semiconductor devices' design processes, allowing faster development cycles and more efficient energy usage.
- Predictive Maintenance: AI technologies can assess the performance and longevity of semiconductor devices in real time, predicting potential failures before they occur.
- Manufacturing Automation: AI enhances automation in semiconductor manufacturing, reducing costs and improving precision through machine learning models tailored to process optimization.
- Supply Chain Management: AI aids in managing complex supply chains, improving logistics and reducing bottlenecks significantly.
In summary, the interplay of AI and semiconductors will likely streamline operations and foster innovation, setting the stage for next-generation technology.
Ending
The conclusion serves as a capstone for the exploration of electric semiconductors. It is a vital section that distills the vast information previously discussed, ensuring that readers grasp the relevance and implications of this subject. This article sheds light on various principles, applications, and innovations surrounding semiconductors, which are crucial in today's technological landscape.
Summary of Findings
Throughout the article, we have dissected several critical aspects of electric semiconductors. Key findings include the understanding of semiconductor materials, their behavior through band theory, and the distinction between intrinsic and extrinsic semiconductors. We examined the manufacturing processes which structure the foundation of technology, focusing on doping methods and crystal growth techniques. The various applications in electronics, telecommunications, and renewable energy are illustrative of the broad impact of semiconductors in modern society.
These findings underscore not only the versatility of electric semiconductors but also their centrality in driving advancements across different sectors. As technology evolves, the importance of these materials only becomes more pronounced, highlighting their role in shaping the future of electronics.
The Significance of Continued Innovation
The semiconductor industry is at the forefront of technological progress. Continued innovation is indispensable to overcome current limitations and expand the potential uses of these materials. Research in nanotechnology, quantum dots, and two-dimensional materials shows promise for advancing how we think about semiconductor applications.
Innovation leads to stronger, more efficient devices. As we focus on environmental concerns and sustainability, the development of new materials and methods can provide more eco-friendly solutions. The future of electric semiconductors will shape not just consumer electronics but also critical infrastructural advancements globally.