Understanding mRNA Sequence Extraction from DNA
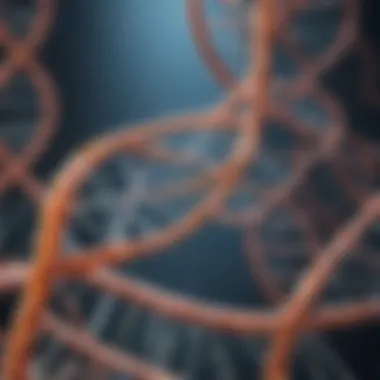
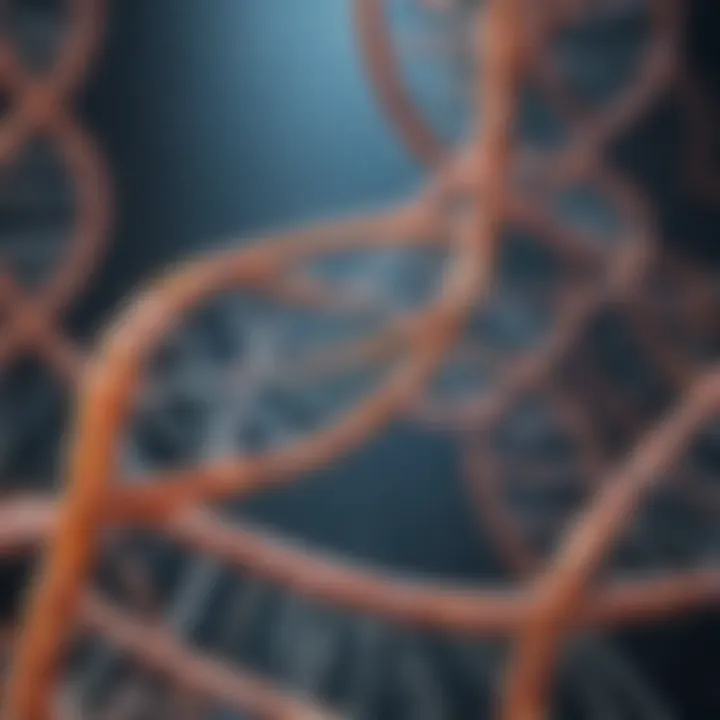
Intro
Transcription, the process of converting DNA into messenger RNA (mRNA), is a fundamental aspect of molecular biology. It's the key to understanding how genetic information is expressed in living organisms. Deciphering the mRNA sequence from its DNA template offers insights into the orchestration of life and the regulation of gene expression. This journey into mRNA synthesis is not just a scientific endeavor; it's a pathway that leads to greater understanding of genetics, disease mechanisms, and potential therapeutic avenues.
Molecular biology is often described as the bridge between genetics and the biochemical processes within a cell. At the heart of this field lies transcription, where the information coded in DNA is transcribed into mRNA. This conversion is crucial because mRNA serves as a template for protein synthesis; thus, comprehending how to accurately deduce the mRNA sequence from DNA is essential for biological research, genetic engineering, and therapeutic applications.
Let’s explore what forms the backbone of this intricate process.
Preamble to DNA and mRNA
Understanding the relationship between DNA and mRNA is essential for grasping the fundamentals of molecular biology. The process by which genetic information is transcribed and translated ultimately governs all biological functions. In this article, we will explore the nuanced workings of DNA and mRNA, focusing on their definitions, roles, and interactions within the complex framework of protein synthesis.
Fundamental Definitions
Overview of DNA
DNA, or deoxyribonucleic acid, serves as the blueprints for all living organisms. It is structured as a double helix, made up of four nucleotide bases: adenine, thymine, cytosine, and guanine. What makes DNA particularly significant in our narrative is its role as the storage house of genetic information. Each strand of DNA carries the instructions necessary to produce everything from proteins to entire organisms.
A remarkable characteristic of DNA is its ability to replicate, allowing genetic information to be passed down through generations. This capability is not only advantageous for continuity but also crucial for addressing genetic diseases through inheritance. However, the rigidity of DNA, as it remains in the nucleus, implies it cannot directly participate in protein synthesis. Therefore, understanding DNA is foundational but must be coupled with the knowledge of mRNA to appreciate the whole picture.
Understanding mRNA
mRNA, or messenger ribonucleic acid, is the dynamic counterpart to DNA. It plays a pivotal role in translating the genetic code stored within DNA into functional proteins that are essential for the cell's operation. The unique feature of mRNA lies in its single-stranded form, which allows it to travel from the nucleus to the cytoplasm where translation occurs.
A key characteristic of mRNA is its ability to undergo modifications, such as the addition of a 5' cap and a poly-A tail, which enhance its stability and translational efficiency. This adaptability makes mRNA not only beneficial for conveying genetic instructions but also a target for advanced therapeutic strategies, like mRNA vaccines. However, the transient nature of mRNA also presents challenges in ensuring the fidelity and timing of protein synthesis across various cellular processes.
The Role of mRNA in Protein Synthesis
Transcription Process
The transcription process is the nuanced orchestration where DNA is converted into mRNA. This vital step involves RNA polymerase, an enzyme that unwinds the DNA double helix and synthesizes the mRNA strand by adding corresponding RNA nucleotides. A critical aspect of this process is the promoter region of DNA, which signals the beginning of gene transcription. The ability of mRNA to faithfully reflect the sequence of the DNA template is paramount; errors here can lead to malfunctioning proteins, which may disrupt cellular functions.
Additionally, transcription factors and enhancers play significant roles in regulating the transcription process, further emphasizing the complexity of genetic expression.
Translation Mechanism
Following transcription, the mRNA must undergo translation, a highly intricate mechanism converting mRNA sequences into amino acids—the building blocks of proteins. Ribosomes, the cellular machinery responsible for translation, read the mRNA in sets of three nucleotides called codons, each codon corresponding to a specific amino acid. This specificity ensures that the protein is synthesized correctly according to the original DNA sequence.
One noteworthy feature of translation is its phase of initiation. Factors such as the presence of initiation factors and various RNA molecules guide the ribosomes to correctly orient with the mRNA. While the translation mechanism is fundamentally robust, it is also subject to errors and regulatory influences that can affect cellular responses.
"The journey from DNA to mRNA and then to proteins is not just a scientific process but a fundamental narrative of life itself."
By laying out these foundational concepts regarding DNA and mRNA, we delve deeper into the overarching mechanisms, techniques, and implications of deciphering mRNA sequences from DNA, providing insights critical for students, researchers, and educators in the field.
The Relationship Between DNA and mRNA
Understanding the relationship between DNA and mRNA is fundamental to grasping the complexities of gene expression and the synthesis of proteins. This connection serves as the backbone of molecular biology, illustrating how genetic information is transcribed and translated into functional units necessary for life. The interplay between these two crucial molecules not only highlights the precision of biological systems but also presents numerous applications in genetic research, medicine, and biotechnology.
The journey begins with the genetic code, the language that DNA uses to orchestrate the production of mRNA. This code consists of sequences of nucleotides grouped into codons, each of which specifies a particular amino acid. Recognizing the molecular mechanics of coding opens doors to understanding how variations in these sequences can lead to different traits or diseases.
Understanding Genetic Code
Codons and Anticodons
Codons and anticodons are integral to the process of translation, the mechanism through which mRNA directs protein synthesis. A codon is a sequence of three nucleotides in mRNA that correlates with a specific amino acid. On the flip side, the anticodon is found on tRNA and is complementary to the codon on the mRNA strand. This complementary interaction is crucial because it ensures the correct amino acid is brought to the growing polypeptide chain. One of the key characteristics of codons is that they are non-overlapping; each nucleotide is part of one codon only, preventing any potential mix-ups during translation. This unique feature minimizes errors in protein synthesis, which can have catastrophic effects on cellular function, exemplifying why codons serve as a reliable choice within molecular biology narratives.
Despite their robustness, there are challenges to consider, such as the implications of synonymous codons – different codons that code for the same amino acid. These variations can affect the efficiency and accuracy of protein production, making this nuanced aspect an important discussion point.
Template and Non-template Strands
The distinction between template and non-template strands is critical when interpreting the dynamics of transcription. The template strand serves as the guide for RNA polymerase to synthesize mRNA, while the non-template strand, often called the sense strand, matches the mRNA sequence (with uracil replacing thymine). This relationship underscores the directional nature of transcription, where synthesis occurs in a 5' to 3' direction, mirroring the complementary nature of DNA and RNA sequences.
One key attribute of the template strand is its specificity for sequence fidelity – the very basis for accurate mRNA synthesis. This property makes it a beneficial concept for understanding how genetic information is conserved and replicated accurately in living organisms. However, while the fidelity of transcription is generally high, it is not infallible.
Complementary Base Pairing
Purines and Pyrimidines
At the core of DNA and RNA structure are the nitrogenous bases classified as purines and pyrimidines. Purines, namely adenine and guanine, consist of a two-ring structure, while pyrimidines – cytosine, thymine (in DNA), and uracil (in RNA) – feature a single-ring configuration. Their pairing plays a critical role in maintaining the integrity of genetic information.
In terms of their pairing, adenine will bond with thymine (or uracil in RNA) and guanine with cytosine, forming stable hydrogen bonds responsible for the double helix structure of DNA. This unique pairing is instrumental, as it facilitates the accurate replication and transcription of genetic material, which is vital to successful gene expression.
However, it’s important to note that the variability in base pair interactions can occasionally lead to mutations or mismatches, which presents challenges to genetic stability.
Pairing Rules
Pairing rules dictate how nucleotides bond within DNA and RNA, ensuring the proper structure and function of these molecules. The rules state that adenine pairs with thymine (and uracil in RNA), and guanine pairs with cytosine. Understanding these rules is crucial for appreciating the underlying mechanisms of DNA replication and RNA transcription.
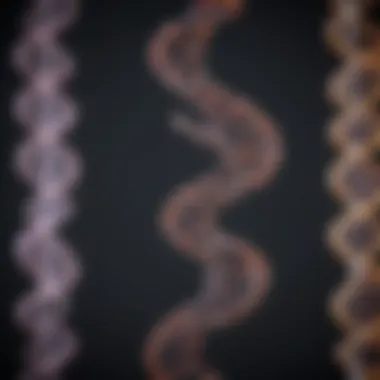
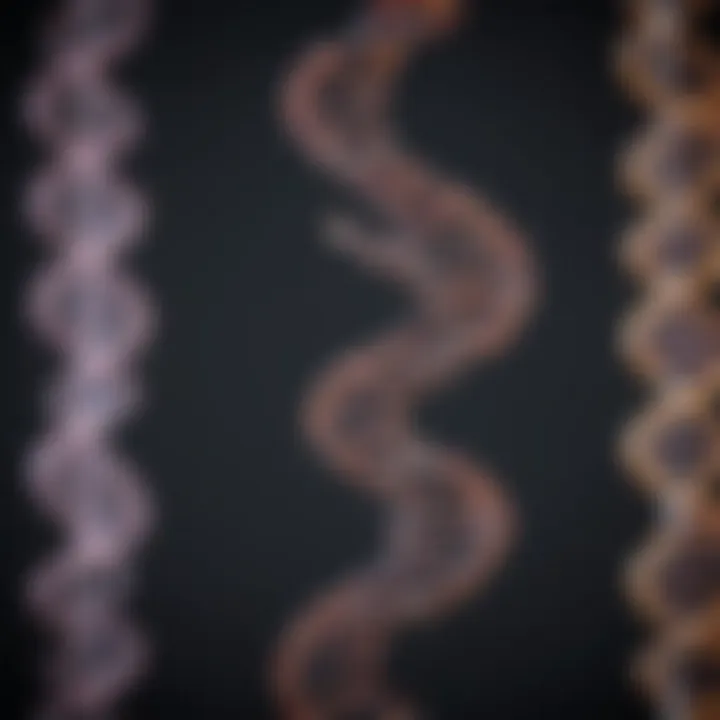
The distinctive characteristic of these pairing rules is their specificity and complementarity, which guarantee that the genetic information is transcribed and accurately maintained. This specificity is a tremendous advantage in the field of genetics, enabling predictive models of gene behavior and mutations. However, mispairing can trigger genetic disorders or anomalies, emphasizing the importance of precise molecular interactions in biological systems.
Overall, the intricate relationship between DNA and mRNA is a testament to the complexity and precision of molecular genetics. By delving into the nuances of genetic code, pairing rules, and the roles of different nucleotide types, we foster a more profound understanding of the processes that sustain life, underscoring the significance of rigorous study and research in this pivotal field.
Transcription: From DNA to mRNA
Transcription is the first crucial step in the overall process of converting DNA into functional mRNA. This essential event serves not only as a bridge but also as a means to express the genetic code stored in DNA. Understanding transcription sheds light on the foundational processes in molecular biology, particularly how genes are expressed in cells. Since mRNA serves as the template for protein synthesis, the mechanisms by which it is formed from DNA are of utmost importance. Capture that moment—transforming a static sequence into a dynamic function—captures the essence of much of what defines life.
Initiation of Transcription
RNA Polymerase Function
RNA polymerase plays a pivotal role in transcription. This enzyme is tasked with synthesizing RNA from a DNA template. Its primary function is to bind to the DNA and unwind the double helix, allowing access to the nucleotides that will form the mRNA strand. One of its most significant characteristics is that it adds nucleotides in a 5’ to 3’ direction, which ensures the correct sequence is built based on the template strand. This function isn't just described in textbooks; its dynamics during initiation are fascinating for various reasons.
The beauty of RNA polymerase lies in its versatility. For example, in eukaryotic cells, there are three different types of RNA polymerases, each responsible for synthesizing different types of RNA. This specialization adds a layer of complexity to the transcription process, allowing for precise regulation. However, this also means that a malfunction in any of these polymerases can lead to significant issues in gene expression.
Promoter Regions
Promoter regions are the landing pads, if you will, for transcription machinery. These specific sequences in the DNA act as essential signals that initiate transcription. Their primary characteristic is that they contain unique sequences, like the TATA box, which are recognized by RNA polymerase and other transcription factors. Without these regions, the whole process of transcription could stall before it begins, highlighting their importance.
The unique feature of promoter regions is their regulatory capability. They can be influenced by various factors, including the presence of enhancers or suppressors, which makes them a point of interest in the study of gene expression. However, the dependence on these sequences also means that slight genetic alterations can disrupt the entire transcription process, leading to potential issues in protein synthesis.
Elongation and Termination
Nucleotide Addition
Once transcription is initiated, the process quickly moves into the elongation phase, where RNA polymerase begins to add nucleotides to form the emerging mRNA strand. This aspect of nucleotide addition is fundamental, as it dictates the mRNA sequence that will ultimately be translated into a protein. A key characteristic of this process is the accuracy required; RNA polymerase has proofreading mechanisms to curb errors during this addition, which is critical in maintaining the fidelity of gene expression.
The unique feature here is that RNA polymerase doesn’t require a primer, unlike DNA polymerase. This means it can initiate synthesis from scratch, which can be both an advantage and a disadvantage. The efficiency of nucleotide addition contributes to the overall speed of transcription but can lead to mistakes if not carefully monitored.
Termination Signals
Termination signals are the cues that bring transcription to a close. These sequences indicate to RNA polymerase that it should stop adding nucleotides and detach from the DNA. The key characteristic of these signals is that they can be formed by specific nucleotide sequences that are recognized by the transcription machinery. Understanding how they function enhances our comprehension of the entire transcription process.
The unique feature of these termination signals is their variability; they can exist in multiple forms across species, complicating the picture when comparing transcriptional regulation. However, the presence of reliable termination signals ensures that transcription halts at the correct length, which is crucial for producing a functional mRNA molecule.
Understanding the intricate details of transcription can illuminate many biological processes, connecting the dots between DNA, RNA, and protein function.
All in all, transcription is an essential part of gene expression. Each step, from initiation to termination, plays a role in ensuring that genetic information is accurately transferred from DNA to mRNA. As science continues to explore these processes, the implications for research in genetics, biotechnology, and medicine are potently significant.
Techniques for mRNA Sequence Determination
Understanding how to determine the mRNA sequence from DNA is central to modern molecular biology and genetic research. Various methods exist to carry out this complex task, each with its own merits and limitations. In this section, we’ll explore different techniques for mRNA sequence determination, focusing particularly on how they contribute to the overall understanding of genetic transcription and expression.
Traditional Methods
Sanger Sequencing
Sanger sequencing, also known as chain termination method, has long been a cornerstone of genetic analysis. This technique involves enzymatically synthesizing DNA while incorporating labeled dideoxynucleotides. Its contribution to mRNA sequence determination hinges on its high accuracy. This method serves well in scenarios where only small segments of DNA or RNA need to be sequenced.
A notable characteristic of Sanger sequencing is its ability to produce long read lengths, which allows researchers to easily identify mutations or variations in specific genes. This makes it a beneficial choice for tasks requiring detailed characterizations, such as examining single-nucleotide polymorphisms (SNPs).
While the method is reliable, it is not without downsides. One unique feature is that it is labor-intensive and time-consuming, making it less suited for large-scale sequencing projects. Thus, while it boasts considerable accuracy, the throughput is low relative to more modern techniques.
Gel Electrophoresis
Gel electrophoresis is a technique that has been around for decades, primarily used for the separation of nucleic acids based on size. This method plays a pivotal role in the analysis of mRNA once it has been extracted, allowing researchers to assess the integrity and size of the RNA fragments.
The key characteristic of gel electrophoresis is its straightforward and visual means of screening RNA samples. It provides a clear representation, allowing experts to evaluate sample quality before proceeding with sequencing methods. This method is beneficial when verifying that the appropriate sizes of the mRNA transcripts are present, which is crucial for downstream applications.
However, this technique is mostly qualitative rather than quantitative. A unique feature is that gel electrophoresis cannot accurately determine the sequence of the RNA itself. Thus, while it serves as a reliable preliminary step in mRNA analysis, it must be complemented with other methods for comprehensive sequencing.
Next-Generation Sequencing Technologies
Illumina Sequencing
Illumina sequencing has transformed the field of genomic research with its high throughput and cost-effectiveness. This method employs a sequencing-by-synthesis technology, allowing for massive parallel sequencing of multiple mRNA samples simultaneously.
The key characteristic of Illumina sequencing is its ability to generate millions of short reads in a single run. This feature leads to an incredibly detailed overview of gene expression profiles, which is crucial for identifying variations and alterations associated with different biological states.
The primary advantage of this technology lies in its scalability, making it a popular choice for large transcriptomics studies. However, short reads can present challenges in terms of alignment and assembly, especially in repetitive regions of the genome.
Pyrosequencing
Another notable method is 454 pyrosequencing, which employs a sequencing-by-synthesis approach, similar to Illumina but with a different chemistry that allows for longer reads.
Its key characteristic is the ability to read a longer sequence of nucleotides than most other next-generation methods. This is particularly useful in identifying gene variants that are larger in scope, providing a more complete picture of the gene's architecture.
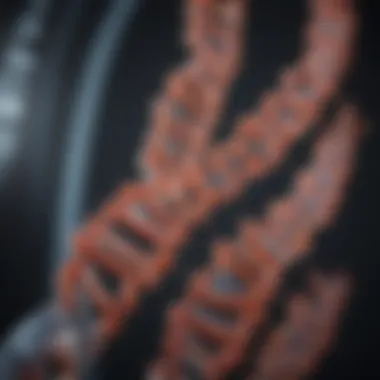
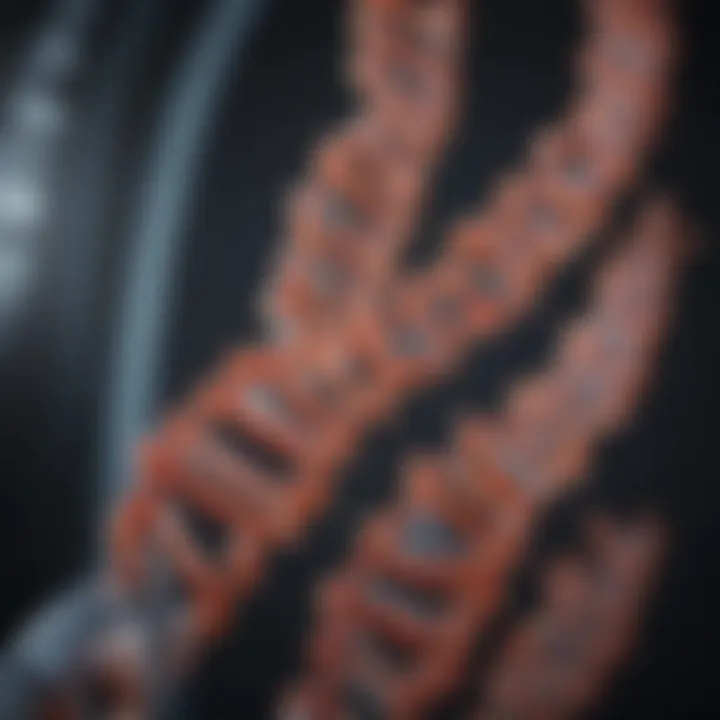
While 454 pyrosequencing has advantages, one significant disadvantage is its higher error rates compared to Illumina sequencing. Furthermore, it has generally lower throughput, which may limit its applications in broader population studies. Nonetheless, its specific application in detailed mRNA sequence determination remains invaluable.
Next-generation sequencing technologies have dramatically expanded our capabilities in understanding gene expression and regulation, marking a significant leap from traditional approaches to modern genomics.
Bioinformatics and mRNA Analysis
Bioinformatics has emerged as a crucial field in understanding the complexities of biological data, specifically in the area of mRNA analysis. In the context of deciphering mRNA sequences from DNA, bioinformatics plays a multi-faceted role by providing tools and approaches to analyze, interpret, and visualize the vast amounts of data generated during genetic studies. Its integration into molecular biology not only enhances the efficiency of data handling but also provides insights that are fundamental in various research fields, including genetics and genomics.
The benefits of bioinformatics in mRNA analysis cannot be overstated. By utilizing computational tools, researchers can address challenges such as data redundancy, inconsistencies, and large-scale analyses that would be impractical through traditional methods. Furthermore, as sequencing technologies evolve and expand in capability, the need for effective bioinformatics practices grows even more critical, ensuring researchers can keep pace with their data requirements.
Software Tools for Sequence Analysis
BLAST
BLAST, or Basic Local Alignment Search Tool, is a widely used algorithm for comparing an amino acid or nucleotide sequence against a database of sequences. Its significance lies in its ability to rapidly find regions of similarity, making it an invaluable asset in the field of bioinformatics and mRNA analysis. The main characteristic of BLAST is its speed and efficiency, allowing users to retrieve relevant sequences quickly without wading through a sea of information.
One notable benefit of BLAST is its user-friendliness coupled with powerful functionalities. Most researchers, whether in academic or clinical settings, can easily navigate and implement it to identify potential homologies, or conserved sequences, between different mRNA strands. However, a potential downside is that while BLAST can identify sequences, it does not provide information about the functional impact of these sequences without further analysis.
Genome Browsers
Genome browsers offer a visual interface for exploring the structure and function of genomes. They compile massive datasets and display them in an easily navigable format that allows researchers to view genes, regulatory elements, and other genomic features. This visualization aspect is pivotal for mRNA analysis as it helps in understanding the context of a specific mRNA within its genomic setting.
The key characteristic of genome browsers is their ability to integrate various data types, such as gene annotations, expression data, and comparative genomics. Thus, they facilitate a holistic view of the genetic architecture. Tools like UCSC Genome Browser and Ensembl are popular among scientists for their robust datasets and comprehensive tools. However, a limitation might be the steep learning curve associated for beginners, where users may initially struggle to extract meaningful insights from complex data.
Data Interpretation
Data interpretation is a critical step resulting from both software analyses and experimental findings. Within this realm, mRNA sequence alignment and functional annotation emerge as vital components that allow researchers to make sense of their data.
Sequence Alignment
Sequence alignment is the process of arranging sequences of DNA, RNA, or proteins to identify similarities and differences. It greatly contributes to mRNA analysis by allowing researchers to compare mRNA sequences across different species or conditions and ascertain evolutionary relationships. Its ability to reveal conserved sequences and mutations provides fundamental insights into gene function, expression, and regulation.
A key characteristic of sequence alignment is its capacity to facilitate comparative analysis in which multiple sequences can be evaluated simultaneously. This broadens the understanding of mRNA functions and their alterations in various biological contexts. Nevertheless, challenges exist, particularly in managing errors that may arise from sequencing technology, which could lead to misalignment and misinterpretation.
Functional Annotation
Functional annotation is the process of linking sequence data to biological meaning. In the context of mRNA analysis, it involves identifying the roles of various mRNA transcripts in cellular processes. By integrating diverse datasets, functional annotation aids in predicting gene functions, pathway participation, and involvement in diseases.
The unique feature of functional annotation is its ability to combine experimental data with computational predictions to enrich the analyses. This attribute makes it especially beneficial for researchers in uncovering novel insights about mRNA transcripts in health and disease. Nonetheless, the reliance on established databases may present discrepancies or outdated annotations, which could misguide interpretations.
In summary, bioinformatics tools and data interpretation strategies are essential components of effective mRNA sequencing identification. By enhancing the accuracy and efficiency of data analysis, they are laying the groundwork for advancements in genetic research.
Challenges in mRNA Sequence Identification
Understanding the mRNA sequence identification process goes hand in hand with grasping the challenges it presents. As researchers dissect the complexity of gene expression and strive for accuracy in sequencing, they must contend with a handful of significant hurdles. The nature of mRNA itself can be both a boon and a bane. For instance, variability in gene expression and the technical aspects of sequencing can present obstacles that complicate analyses. Navigating these challenges demands not only knowledge but also innovative methodologies.
Variability in Gene Expression
Alternative Splicing
Alternative splicing is a fascinating aspect of mRNA processing that generates multiple mRNA variants from a single gene. This process allows for the production of different protein isoforms, which might play unique roles within the cell. One key characteristic of alternative splicing is its ability to expand the functional repertoire of the genome without the need for additional genes. The intricacies of this process can lead to various misunderstandings during mRNA sequencing. For example, if a sequencing method does not account for specific splice variants, it may result in incomplete or misleading data.
This unpredictability showcases why alternative splicing is both beneficial and challenging in mRNA sequence identification. On one hand, it broadens the biological functionality; on the other, it complicates how we interpret rich datasets derived from RNA experiments. The unique feature of alternative splicing is its dynamic nature, allowing for differential expression of proteins under various conditions, which could be an advantage in discovering disease markers but can also muddle analyses by introducing variable results.
Post-transcriptional Modifications
Post-transcriptional modifications include a range of processes such as methylation, polyadenylation, and RNA editing. These modifications significantly impact mRNA stability, localization, and translation efficiency. A key characteristic of post-transcriptional modifications is that they can influence gene expression levels and protein output, thus playing a crucial role in cellular function.
In discussions of mRNA sequencing, these modifications can be incredibly beneficial, as they can provide insights into regulatory mechanisms. However, the presence of these modifications complicates sequencing efforts. A unique feature of these alterations is that their occurrence varies among cell types and environmental conditions. This variability presents both advantages, such as potential insights into cellular responses, and disadvantages, including the challenge of accurately identifying modified RNA species during sequencing.
Technical Limitations of Sequencing
Errors in Sequencing
Errors in sequencing are a common drawback of genetic analysis. These errors can happen due to several reasons, including low-quality templates and limitations in sequencing technologies. Notably, sequencing errors can lead to misinterpretation of the mRNA sequences, resulting in a cascade of inaccuracies in downstream analyses. One pivotal point about these errors is how they can propagate through an experiment, leading to invalid conclusions about gene expression.
So, why is discussing sequencing errors crucial in this article? Because understanding their root causes can guide researchers towards better methodologies and more accurate interpretations of their data. The unique aspect of sequencing errors lies in their potential occurrence during both the generation of raw data and subsequent analysis stages, thus emphasizing the need for stringent quality control measures.
Short Reads vs. Long Reads
The debate between short reads and long reads in sequencing has significant implications for mRNA identification. Short reads facilitate rapid sequencing and cost-effectiveness, but they are often limited in their ability to span entire transcripts or complex regions containing extensive alternative splicing. Conversely, long reads provide a comprehensive picture of the transcriptome, allowing for better resolution of splicing events and complete isoforms.
Choosing between short reads and long reads involves weighing the pros and cons of each technology. Short reads might be viewed as a default choice due to their speed and lower cost; however, the advantage of long reads in capturing the full length and context of mRNA is undeniable. The unique feature of long-read sequencing is its potential to reduce ambiguities in gene annotations, yet these technologies can be more expensive and require complex instrumentation.
In summary, navigating the challenges in mRNA sequence identification requires a keen understanding of both biological variability and sequencing technologies. Addressing these hurdles not only enhances the integrity of the data but also propels the field of molecular biology forward.
Applications of mRNA Sequencing
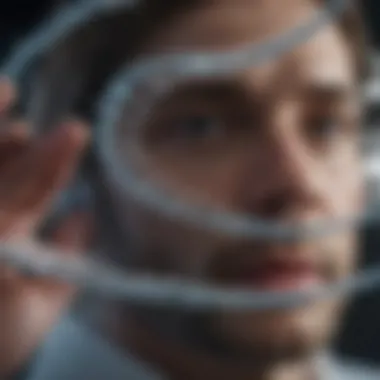
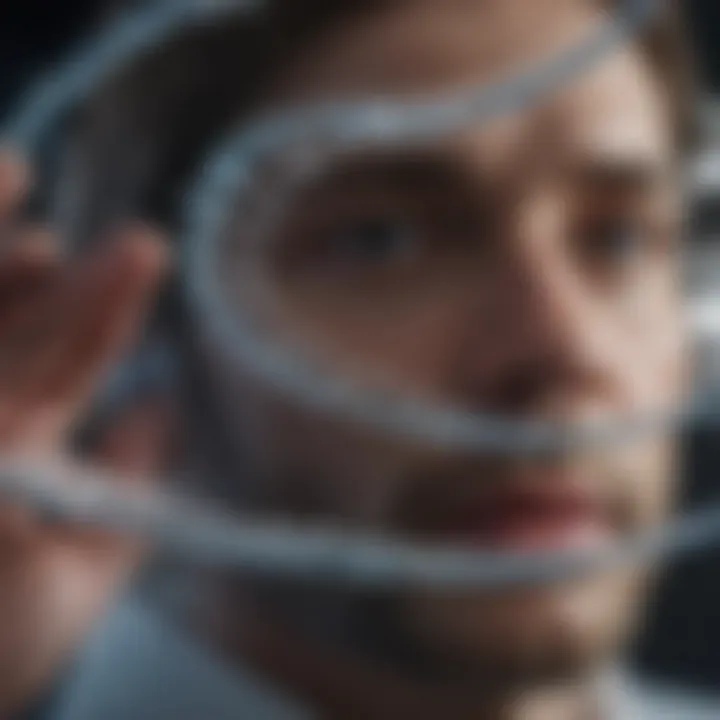
mRNA sequencing, often referred to simply as RNA-Seq, stands at the forefront of modern molecular biology. Its applications range from disease diagnostics to therapeutic advancements, making it an indispensable tool in research and clinical settings. By translating the genetic code stored in DNA into functional proteins via mRNA, scientists can investigate cellular processes and disease mechanisms with unparalleled precision. This section will delve into two primary areas where mRNA sequencing has made an impact: medical diagnostics and therapeutic development.
Medical Diagnostics
RNA-Seq in Cancer Research
RNA-Seq has revolutionized cancer research by enabling in-depth exploration of gene expression patterns in tumors. One specific aspect of this technique is its ability to detect abnormal gene activity, which is crucial for understanding how cancers develop and progress. This high-throughput approach not only identifies previously known oncogenes but can also reveal new targets for therapy or prognosis.
The key characteristic of RNA-Seq in cancer research is its sensitivity, allowing for the detection of low-abundance transcripts that traditional methods might miss. As a result, this method has become a favorite among researchers aiming to dissect the complexities of tumor biology. The unique feature here lies in its capacity to provide a comprehensive snapshot of the transcriptome, which includes not just protein-coding genes but also non-coding RNAs that may play significant roles in cancer development.
However, there are some disadvantages to consider. The sheer volume of data generated demands substantial computational power and expertise for analysis, and the costs can add up quickly. Despite these challenges, RNA-Seq remains a powerful approach in the quest for personalized cancer treatments, improving patient outcomes through tailored therapies.
Disease Biomarkers
When it comes to identifying disease biomarkers, RNA-Seq shines by uncovering unique expression signatures linked to various conditions. Biomarkers are vital indicators of disease presence or progression, and their identification through mRNA sequencing can lead to earlier diagnosis and better monitoring of diseases.
A notable characteristic of disease biomarkers identified via mRNA sequencing is their specificity. Certain mRNA profiles can distinguish between healthy and diseased states, enabling clinicians to make more informed treatment decisions. The unique aspect here is the potential for developing non-invasive tests, which can utilize biological samples like blood or saliva instead of tissue biopsies.
However, while the promise of RNA-Seq in finding biomarkers is significant, it isn’t without drawbacks. Method reproducibility and biological variability can create hurdles in validating these biomarkers across diverse populations. Nonetheless, the pursuit for reliable disease indicators continues, driven by the capabilities of mRNA sequencing.
Therapeutic Development
Therapeutic development has also benefited immensely from mRNA sequencing. The insights gained from understanding gene expression are instrumental in developing novel treatments and strategies to combat various diseases.
Gene Therapy Approaches
Gene therapy approaches harness the therapeutic potential of RNA, where treatments involve introducing, removing, or altering genetic material. A specific aspect of gene therapy that stands out is its personalized nature; RNA-Seq can guide the design of therapies tailored to an individual's genetic profile. This adaptability makes the methodology popular among researchers and clinicians alike.
One key characteristic of gene therapy is its targeted approach. By understanding the specific RNA profiles of a patient’s diseased cells, researchers can pinpoint the precise modifications needed to restore normal function, greatly enhancing the effectiveness of treatments. However, a unique feature comes with the challenges of delivery systems and potential immune responses. These factors can complicate the implementation of gene therapies, but ongoing research seeks to address these hurdles for successful applications.
Vaccination Strategies
Vaccination strategies have also seen a shift due to advancements in mRNA technologies. One fascinating aspect is the use of mRNA as a platform for creating vaccines that instruct cells to produce proteins that mimic viral components, stimulating an immune response.
The key characteristic of mRNA vaccines is their speed and adaptability. Once the genetic sequence of a pathogen is determined, researchers can rapidly develop mRNA vaccines to counteract infections, as seen with recent COVID-19 vaccines. This unique feature highlights the advantages of using mRNA over traditional vaccines, which often require cultured virus or protein production.
Nonetheless, mRNA vaccines come with their own set of challenges, including stability and the need for cold storage, which can complicate distribution efforts. Despite these limitations, the success of mRNA technologies in vaccination has opened doors to a new era in preventive medicine, paving the way for future developments.
In summary, the applications of mRNA sequencing span a multitude of grounds within both medical diagnostics and therapeutic development. From uncovering cancer pathways and identifying disease biomarkers to revolutionizing treatment strategies through gene therapy and vaccination, the insights gained from mRNA sequencing present a potent avenue for progress in health and therapeutics.
Future Directions in mRNA Research
Research into mRNA has taken leaps and bounds in recent years, making it a hot topic in the scientific realm. As we delve deeper into this article, the focus on future directions isn't just about what's next; it involves understanding how emerging technologies and ethical considerations can reshape our approach to mRNA research.
Emerging Technologies
Single-Cell RNA Sequencing
Single-cell RNA sequencing (scRNA-seq) is a game-changer in the field of genetics. It allows researchers to examine the activity of individual cells, instead of averaging signals from a population of cells. This technique can illuminate the complexities of cellular heterogeneity, uncovering insights into how mRNA variations contribute to differences in cell function and behavior.
One of the key characteristics of scRNA-seq is its ability to profile thousands of individual cells simultaneously, providing a high-resolution view of gene expression. This fine-tuning of data offers a new lens through which we can observe the intricate details of cellular environments, making it a popular choice among researchers aiming for precision.
A unique aspect of single-cell RNA sequencing is its capacity to track dynamic changes over time. This feature allows scientists to monitor developmental processes and responses to stimuli at an unprecedented depth. However, the technology is not without its hiccups—it can be costly and demands a solid grasp of bioinformatics, making it a double-edged sword in certain experimental contexts.
Nanopore Sequencing
Nanopore sequencing, on the other hand, is revolutionizing the scalability of sequencing techniques. This method involves threading DNA or RNA molecules through a tiny protein-based pore and measuring changes in electrical current to determine the sequence. The main draw of nanopore sequencing is its ability to read long sequences, thereby enabling researchers to capture complete transcripts in a single run.
The key characteristic that makes nanopore sequencing beneficial is its real-time data generation, providing immediate results that can be critical for time-sensitive research. This speed allows for swift adjustments or further studies while the research is ongoing. Moreover, its portability is truly appealing—scientists can conduct sequencing in remote locations, which can be a game changer for field studies.
Nevertheless, it has drawbacks as well. The error rates can be high compared to other sequencing technologies, which can complicate data analysis. But the advantages of speed and flexibility often outweigh these issues, making it a vital tool for the future of mRNA research.
Ethical Considerations
Genome Editing Regulations
As we charge ahead with mRNA technologies, genome editing regulations loom large in the discussion. These regulations ensure that as scientists explore the potential of mRNA for applications like gene editing, they do so within an ethical framework. The importance of these regulations cannot be overstated, as they protect not only human health but also biodiversity.
One defining feature of genome editing regulations is the balance they strike between innovation and caution. These rules allow researchers to push the boundaries of science while maintaining safety and ethical standards. Considering the rapid pace of advancements, the regulations also adapt to new findings, ensuring that they remain relevant in an ever-evolving field.
However, some argue that overly stringent regulations may stifle scientific progress, creating a dilemma that researchers must navigate. Nonetheless, they play a crucial role in shaping the responsible use of technology, keeping the pursuit of knowledge grounded in ethical practice.
Privacy in Genetic Data
Amid the advances in mRNA research, privacy concerning genetic data also becomes an essential topic. With the increasing ability to sequence and analyze genetic material, concerns over who has access to this information are at an all-time high. Protecting individuals' genetic data is vital not just for the integrity of research but for personal rights as well.
A noteworthy characteristic of privacy regulations in genetic data is their focus on informed consent. Researchers must ensure that individuals providing their genetic information understand how it will be used. This insistence on transparency serves to build trust between the scientific community and the public.
However, challenges persist. As technology evolves, so do methods of data handling and storage, which may put sensitive information at risk. Moreover, the potential for genetic data misuse, whether for discrimination or other unethical purposes, underscores the necessity of stringent privacy measures. Yet, despite these concerns, establishing robust privacy standards is paramount to advancing mRNA research with ethical integrity.
"In the race toward innovation, the balance of ethics and progress must be carefully maintained to ensure beneficial outcomes for society."
The future of mRNA research looks promising, but it comes with responsibilities that scientists must confront head-on. The interplay between cutting-edge technologies and ethical considerations will shape the next waves of discovery.