Understanding DNA's Role in Protein Synthesis
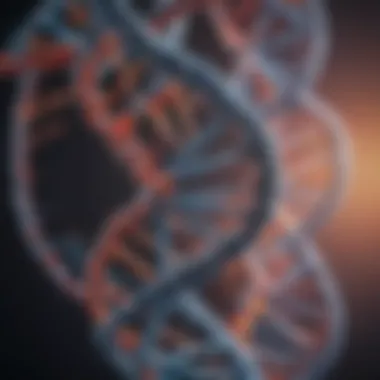
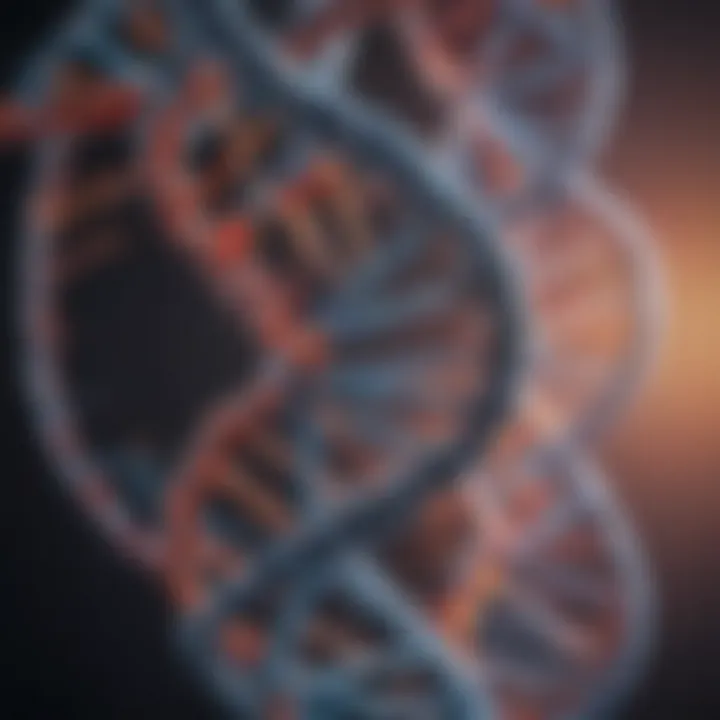
Intro
The process by which DNA translates into protein synthesis is fundamental to all living organisms. Understanding this mechanism is essential not just for biologists but also for anyone interested in genetics, biotechnology, and molecular biology. This article dissects the complexities of protein synthesis, focusing on the interplay between DNA, RNA, and ribosomes, crucial players in the central dogma of molecular biology.
Research Overview
In this section, we summarize the main findings and the significance of the mechanisms involved in protein synthesis. The conversion of genetic information into functional proteins involves several key steps: transcription and translation. Each step has distinct roles played by different molecules that interact with one another in a highly regulated manner. Evaluating these processes provides insights into how genetic information is expressed and how proteins are synthesized to perform countless functions within an organism.
Summary of Key Findings
- Structure of DNA: DNA serves as the blueprint for all cellular functions. Its double-helix structure encapsulates sequences called genes, which code for proteins.
- Transcription Process: This is the first step in protein synthesis, where the genetic code in DNA is transcribed to produce messenger RNA (mRNA).
- Translation Process: Following transcription, mRNA is translated into protein within the ribosomes. The roles of transfer RNA (tRNA) and ribosomal RNA (rRNA) become vital here as they help decode the mRNA sequence into amino acids, forming proteins.
Importance of the Research
The significance of studying protein synthesis extends beyond academic interest; it has practical applications in fields like medicine, agriculture, and genetic engineering. For example, understanding how proteins are created can lead to advances in gene therapy, the production of pharmaceuticals, and the enhancement of crops. By knowing the intricacies of the transcription and translation processes, researchers can manipulate these pathways to achieve desired outcomes.
Methodology
The exploration of these mechanisms relies on a robust methodology that combines theoretical frameworks with empirical studies.
Study Design
The study design encompasses molecular analysis, biochemical experiments, and genetic studies to unravel the steps of protein synthesis. Various organisms may be studied to observe both similarities and unique adaptations in their protein-making processes.
Data Collection Techniques
Data is typically collected through experimentation that measures the efficiency and accuracy of transcription and translation. Techniques involved may include:
- Polymerase Chain Reaction (PCR): Used to amplify DNA sequences for analysis.
- Western Blotting: This allows researchers to visualize specific proteins in a sample.
- RNA Sequencing: This technique maps out RNA presence and abundance, clarifying which genes are activated under certain conditions.
The Role of DNA in Cells
DNA plays a crucial role in the life of each cell. It serves as the instruction manual for building and maintaining an organism. Understanding this role is foundational to grasp how genetic information translates into functional proteins. This section explores how DNA functions in cells, its structure, and its profound significance in genetic coding and protein synthesis.
Basic Structure of DNA
DNA, or deoxyribonucleic acid, has a unique double-helix structure. This shape is formed by two long strands of nucleotides twisted around each other. Each nucleotide comprises a sugar, phosphate group, and a nitrogenous base. There are four types of nitrogenous bases in DNA: adenine, thymine, cytosine, and guanine. The sequence of these bases encodes genetic information.
Key features include:
- Double helix formation: This design allows DNA to be compact and stable.
- Complementary base pairing: Adenine pairs with thymine, while cytosine pairs with guanine. This pairing is vital for accurate DNA replication.
- Antiparallel strands: The two strands run in opposite directions, facilitating the replication and encoding processes.
This structure enables the essential role of DNA as the carrier of genetic information in cells.
Function of DNA in Genetic Coding
The primary function of DNA is to store and transmit genetic information. Each sequence of bases along the DNA strand corresponds to specific instructions for making proteins.
Key functions include:
- Gene expression: Genes, segments of DNA, are expressed to produce proteins that perform various functions in the body, including structural roles and enzymatic activity.
- Replication: Before a cell divides, its DNA is replicated, ensuring that each new cell has an exact copy of the genetic instructions.
- Mutation: Changes in the DNA sequence can lead to variations, some of which may have beneficial effects, while others can result in diseases.
Understanding how DNA works is essential for comprehending how the genetic code determines the traits and functions of living organisms. Through studying this subject, researchers can manipulate genetic material in fields like biotechnology and medicine.
Understanding Protein Synthesis
Understanding protein synthesis is integral to comprehending how genetic information is transformed into functional components of life. Proteins serve as the building blocks for various biological structures and functions. The process involves two main stages: transcription and translation, which convert DNA codes into proteins. Each step is characterized by specific interactions and enzymatic activities that ensure accuracy and efficiency. By studying protein synthesis, we gain insights into cellular functions, hormonal responses, and the mechanisms of disease.
Overview of Protein Functionality
Proteins are incredibly diverse in function. They act as enzymes, antibodies, transport molecules, and structural components in cells. Each protein's functionality is determined by its amino acid sequence, which is dictated by the underlying DNA sequence. This direct relationship emphasizes the importance of DNA in coding these complex structures.
Every protein performs biochemical tasks that are critical for maintaining homeostasis within an organism. For example, enzymes catalyze biochemical reactions, while structural proteins provide support for cellular architecture. The specific shapes and chemical properties of proteins allow them to perform their functions effectively. Overall, understanding how proteins function is essential for grasping biological systems.
Importance of Proteins in Biological Systems
Proteins play a crucial role in numerous biological processes. Here are some key areas where proteins are essential:
- Catalysis: Enzymes accelerate chemical reactions that are vital for metabolism.
- Defense: Antibodies are proteins that protect against pathogens.
- Transport: Hemoglobin, a protein in red blood cells, transports oxygen.
- Structure: Collagen provides strength and support to connective tissues.
The significance of proteins extends beyond mere biological functions; they are foundational to cellular communication and signaling pathways. Proteins interact with each other and with nucleic acids to regulate various cellular processes such as gene expression, cell division, and apoptosis.
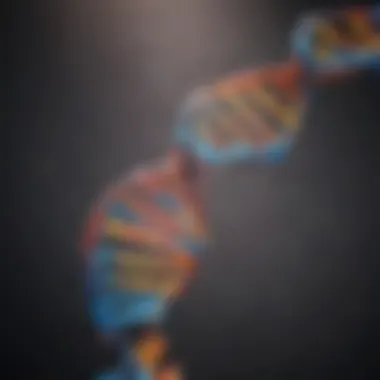
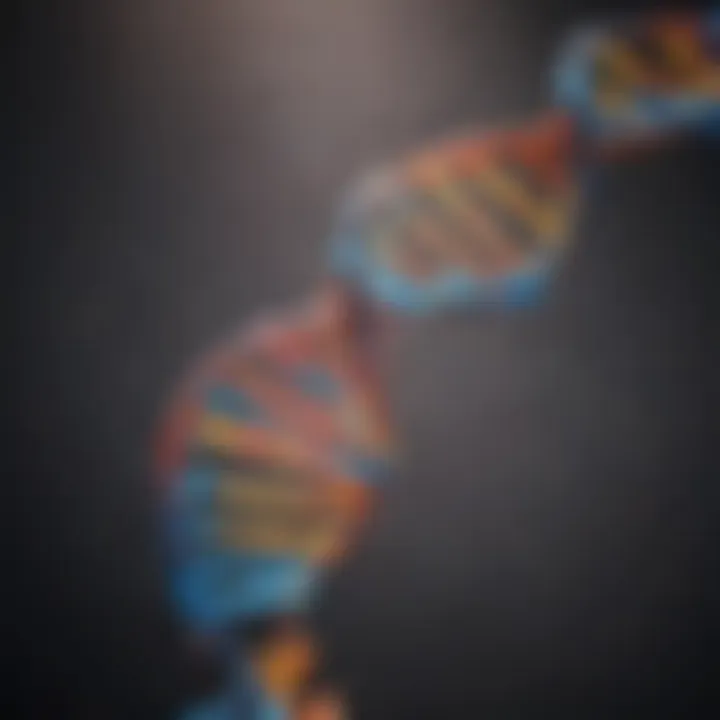
In summary, proteins are indispensable for life. Their diverse functionalities and roles underscore the complexity of biological systems, making the study of protein synthesis invaluable for both scientific research and medical advancements.
"Proteins are not just the building blocks of life; they are the architects and messengers that convey life's blueprint into reality."
By ensuring a deep understanding of how proteins are synthesized and function, researchers and professionals can better address challenges in genetics, biotechnology, and medicine.
The Central Dogma of Molecular Biology
The concept of the central dogma of molecular biology is foundational in understanding how genetic information is utilized within a cell. This framework not only explains the flow of genetic information from DNA to RNA and finally to protein, but it also emphasizes the role of these molecules in cellular function. The central dogma is significant in various fields. It underpins molecular biology, genetics, and even biotechnology. Understanding this process allows researchers to grasp how genes are expressed, regulated, and how they contribute to phenotypes.
Definition of the Central Dogma
The central dogma, first articulated by Francis Crick in 1957, describes the transfer of genetic information from DNA to RNA and then to protein. It is often simplified into the formula: DNA → RNA → Protein. At its core, this definition highlights three major processes: replication, transcription, and translation. Each of these processes plays a crucial role in maintaining the flow of genetic information.
- Replication: The copying of DNA to ensure that genetic information is passed on during cell division.
- Transcription: The process where a specific segment of DNA is transcribed into RNA.
- Translation: The conversion of mRNA sequences into a sequence of amino acids, leading to protein formation.
This dogma illustrates the linear pathway of genetic expression. However, it is essential to note that there are exceptions and complex interactions in actual biological systems, including processes like reverse transcription, where RNA can be converted back to DNA. These nuances reflect the adaptability and intricacy of genetic regulation within living organisms.
Process from DNA to Protein
The progression from DNA to protein is a multi-step process that involves intricate molecular machineries. Each step contributes critically to the final outcome of protein synthesis.
- DNA Replication: Before any protein can be synthesized, the DNA molecule must be replicated. This ensures that each daughter cell receives a complete set of genetic information. Enzymes such as DNA polymerases play a significant role in this replication process.
- Transcription: During transcription, a specific gene is transcribed into messenger RNA (mRNA). This process involves several steps:
- Translation: The synthesized mRNA is then translated into protein. This occurs in several stages:
- Initiation: RNA polymerase binds to a gene's promoter region.
- Elongation: RNA polymerase unwinds the DNA and synthesizes a complementary RNA strand.
- Termination: The process concludes when RNA polymerase reaches a termination signal, leading to the release of the mRNA transcript.
- Initiation: The small ribosomal subunit binds to the mRNA and the first tRNA.
- Elongation: tRNAs bring the appropriate amino acids corresponding to the mRNA codons. The ribosome moves along the mRNA, facilitating the peptide bond formation between amino acids.
- Termination: The process continues until a stop codon is reached, leading to the release of the newly formed protein.
Understanding this entire process is crucial for researchers and professionals working in fields such as genetics, molecular biology, and biochemistry. The central dogma is not merely a theoretical framework but a practical guide to comprehending the mechanisms that drive life at the molecular level.
"The central dogma provides a foundational understanding of genetic expression and cellular function. It symbolizes a critical pathway that fuels biological systems."
This knowledge enables advancements in biotechnological applications, as manipulation of these processes can lead to innovations in therapeutic development, genetic engineering, and more.
Transcription: First Step in Protein Synthesis
Transcription is the first crucial step in protein synthesis, serving as the bridge between the genetic information encoded within DNA and the production of proteins. This process is vital for the expression of genes, as it creates messenger RNA (mRNA) copies of the DNA sequences needed for protein synthesis. Understanding transcription helps clarify how genetic information is translated into functional molecules that perform various roles within cells.
This section highlights the mechanisms of transcription, emphasizing its significance in cellular function and overall biological processes. Efficient transcription ensures that the right proteins are produced at the right times in response to cellular needs. Misregulation of this process can lead to various diseases, making it essential for researchers and educators to comprehend.
Initiation of Transcription
The initiation of transcription is the process where RNA polymerase, a key enzyme, attaches to a specific region of DNA called the promoter. The promoter contains crucial signals that direct RNA polymerase to the correct starting point for transcription. During this phase, several factors called transcription factors assist in the recognition and binding of RNA polymerase to the promoter region. Once properly bound, the DNA strands unwind, creating a transcription bubble that allows the RNA polymerase to access the template strand of DNA.
This step is critical as it determines the specificity and timing of gene expression. Without accurate initiation, downstream processes may face disruptions, leading to protein shortages or overproduction. Thus, understanding how transcription begins is fundamental in the broader context of gene regulation.
Elongation and Termination Phases
After the successful initiation of transcription, the elongation phase begins. During this phase, RNA polymerase moves along the DNA template strand, synthesizing a complementary strand of mRNA in the 5' to 3' direction. The RNA nucleotide Uracil (U) replaces Thymine (T) found in DNA. This elongation continues until RNA polymerase reaches a termination signal within the DNA sequence.
Upon reaching a termination signal, the process concludes. The elongation phase is essential for producing a complete mRNA strand that carries the encoded information from DNA. Any mistakes during elongation can lead to improperly formed proteins, which can negatively affect cellular functions.
In summary, the elongation and termination phases illustrate the precision required during transcription to ensure accurate mRNA production. Both phases emphasize the need for proper regulation and functioning of the transcription machinery.
Role of RNA Polymerase
RNA polymerase plays a pivotal role throughout the transcription process. This enzyme not only facilitates the unwinding of DNA but also catalyzes the formation of the RNA strand. It reads the template DNA strand and adds the appropriate RNA nucleotides during synthesis.
RNA polymerase is essential in detecting the promoter regions, ensuring specificity in gene expression. There are different types of RNA polymerase in eukaryotes, such as RNA polymerase I, II, and III, each responsible for synthesizing different types of RNA.
"The precise function of RNA polymerase can greatly influence gene expression and, consequently, cellular functionality."
Post-Transcriptional Modifications
Post-transcriptional modifications are essential processes that occur after the initial transcription of a gene. These modifications serve to enhance the stability, translation efficiency, and overall function of the messenger RNA (mRNA) molecule. They play a crucial role in ensuring that the genetic information encoded in DNA is accurately translated into functional proteins. Several key elements include the addition of a 5' cap, polyadenylation, and splicing. Each of these processes contributes to the maturation of mRNA before it exits the nucleus and enters the cytoplasm.
Capping and Polyadenylation
Capping refers to the addition of a modified guanine nucleotide at the 5' end of mRNA. This cap protects the mRNA from degradation and facilitates its recognition by the ribosome during translation. The presence of a 5' cap is crucial for the efficient initiation of translation.
Polyadenylation involves adding a string of adenine nucleotides to the 3' end of the mRNA molecule. This poly(A) tail enhances mRNA stability and also plays a role in the regulation of gene expression. Both capping and polyadenylation are vital in maintaining the integrity of the mRNA, ensuring that it survives longer in the cytoplasm to successfully code for proteins.
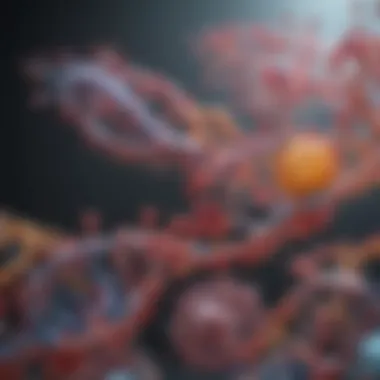
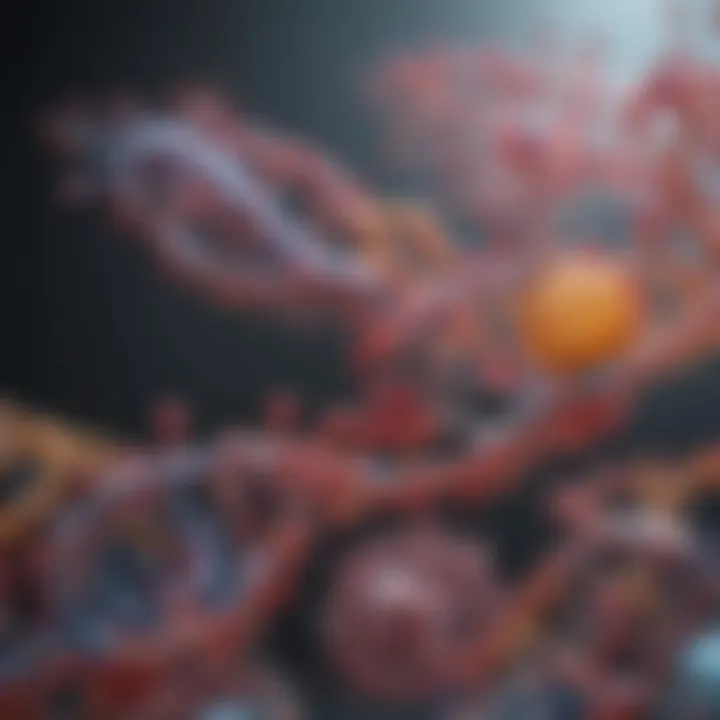
Splicing of Introns and Exons
Splicing is another important post-transcriptional modification. It involves the removal of non-coding regions, known as introns, and the joining together of coding regions, known as exons. During splicing, a spliceosome—a complex of proteins and RNA—recognizes specific sequences at the intron-exon boundaries. The precise removal of introns and subsequent ligation of exons is crucial for producing a functional mRNA transcript.
This process allows for alternative splicing, where different combinations of exons can be included or excluded from the final mRNA molecule. This versatility is beneficial as it enables a single gene to produce multiple protein isoforms. The ability to generate diverse proteins is particularly important for complex organisms, where proteins may have specialized functions.
"Post-transcriptional modifications fine-tune the gene expression process, ensuring that the right proteins are synthesized at the right time and in the appropriate amounts."
In summary, post-transcriptional modifications like capping, polyadenylation, and splicing are fundamental in shaping the fate and functionality of mRNA. They ensure that the genetic message is preserved and effectively translated, bridging the gap between DNA and protein synthesis.
Translation: From RNA to Protein
Translation is essential in the flow of genetic information. It is the process where messenger RNA (mRNA) is converted into a polypeptide chain, ultimately forming a functional protein. This step integrates various cellular components, making it crucial for cellular functions and overall metabolic processes.
In this section, we delve into three key elements of translation: the overall mechanism, the role of ribosomes, and the function of transfer RNA (tRNA). Each of these components plays a vital role in ensuring the accuracy and efficiency of protein synthesis.
Overview of Translation Mechanism
The translation mechanism begins with the assembly of ribosomes around the mRNA template. The ribosome scans the mRNA to identify the start codon, which indicates where translation commences. Once ribosomal subunits are in place, the next step involves the recruitment of tRNA molecules. Each tRNA carries a specific amino acid corresponding to the codon sequence on the mRNA.
During translation, ribosomes move along the mRNA strand, facilitating the sequential addition of amino acids to the growing polypeptide chain. This elongation continues until a stop codon is reached, signaling the termination of the translation process. At this point, the completed polypeptide is released to undergo further folding and modifications.
Role of Ribosomes in Translation
Ribosomes serve as the cellular machinery responsible for translating mRNA into proteins. These complex structures are composed of ribosomal RNA (rRNA) and proteins, arranged into two subunits: the large and small subunits. The assembly of these subunits occurs only during translation, highlighting their functional specificity.
Ribosomes have three binding sites for tRNA: the A (aminoacyl), P (peptidyl), and E (exit) sites. The A site accommodates new tRNA bound to an amino acid, the P site holds the growing polypeptide chain, and the E site is where discharged tRNA exits. This arrangement promotes efficiency, as ribosomes can facilitate the simultaneous binding of tRNA and synthesis of the polypeptide chain.
tRNA and Its Function
Transfer RNA, or tRNA, plays a pivotal role in translation by serving as an adapter between the mRNA codon and the corresponding amino acid. Each tRNA molecule has an anticodon region that pairs with its complementary codon on the mRNA. This ensures that the correct amino acid is added to the polypeptide chain in the right sequence.
The process of tRNA charging is critical for its function. Transfer RNAs become attached to their specific amino acids through an enzyme called aminoacyl-tRNA synthetase. This charging process is crucial, as it dictates the accuracy of translation.
In summary, the translation step reinforces the importance of RNA in protein synthesis. By understanding the translation mechanism, the functional roles of ribosomes, and the nature of tRNA, one can appreciate how complex yet precise the process of synthesizing proteins is within a cell.
Amino Acids and Their Significance
Amino acids form the foundation of protein structures and are vital in many biological processes. They serve as the building blocks for proteins, which play critical roles in cellular function and structure. Understanding amino acids enhances comprehension of how proteins are synthesized from genetic material. Each protein's unique sequence and structure arise from the specific arrangement of amino acids. This makes them essential for functions such as enzyme catalysis, signaling, and structural support in cells.
Building Blocks of Proteins
Proteins are polymers composed of long chains of amino acids. There are twenty standard amino acids encoded by the genetic code. These amino acids link together via peptide bonds, forming a polypeptide chain. Depending on the specific sequence of amino acids, proteins can fold into intricate three-dimensional shapes. This folding is crucial since a protein's function is directly related to its structure. For example:
- Enzymes accelerate biochemical reactions.
- Antibodies play a role in the immune response.
- Transport proteins facilitate the movement of molecules across cell membranes.
The sequence of amino acids in a protein is determined by the order of nucleotides in the corresponding mRNA, reflecting the genetic information transcribed from DNA. Thus, any change in the order can potentially alter protein functionality, contributing to various cellular processes.
The Genetic Code
The genetic code consists of sequences of nucleotides that encode amino acids. It is a triplet code, meaning three nucleotides correspond to one amino acid. For example, the codon AUG codes for methionine, which is also the start signal for protein synthesis. This triplet nature introduces redundancy, as multiple codons can code for the same amino acid. This feature can buffer against mutations that might otherwise harm protein function.
The genetic code is nearly universal across organisms, which emphasizes its significant role in the evolutionary biology of living beings. A thorough grasp of the genetic code is essential for several applications, including genetic engineering and biotechnology, allowing scientists to manipulate sequences for desired protein outcomes.
Understanding amino acids and their roles in the genetic code is fundamental to unraveling the intricate process of protein synthesis, critical for research and application in biotechnology and medicine.
Folding and Modifying Proteins
Folding and modifying proteins is a critical part of understanding how proteins function in biological systems. After synthesis, proteins do not automatically take on their useful forms. They must undergo a series of precise folding and modifications to become biologically active. Misfolded proteins can lead to serious diseases, making this process even more crucial.
Importance of Protein Folding
Protein folding refer to the process where a linear chain of amino acids assumes a specific three-dimensional structure. This final shape determines the protein’s functionality in biological processes. Each protein is like a unique puzzle; it only works when every piece fits perfectly.
Key aspects of protein folding include:
- Correct Functionality: A protein can only perform its role effectively when folded correctly. For example, enzymes need their active sites to be exposed for catalysis.
- Prevention of Diseases: Misfolding can result in conditions such as Alzheimer’s or Cystic Fibrosis. Understanding how proteins fold can aid in discovering treatments for these diseases.
- Chaperone Proteins: These specialized proteins assist in the folding process, ensuring that proteins achieve their proper shapes without forming aggregates.
Post-Translational Modifications
Once proteins have folded, many undergo additional changes known as post-translational modifications (PTMs). These modifications fine-tune protein function and activity. PTMs can change the protein’s shape, localization, stability, and activity.
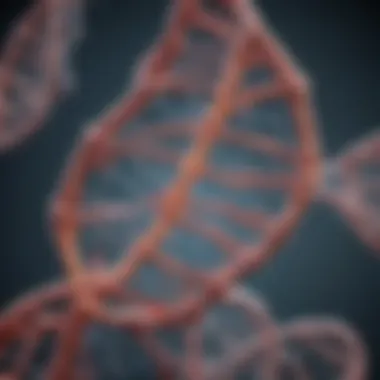
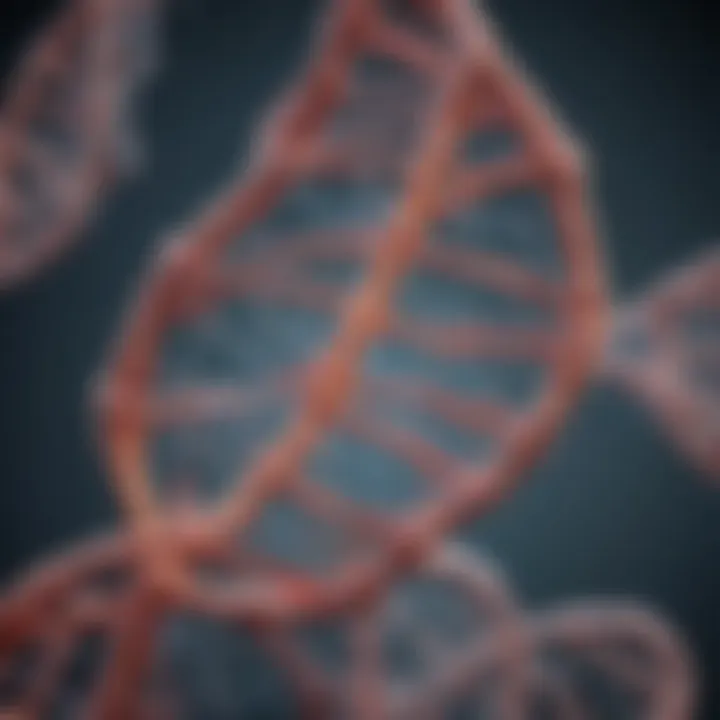
Common types of PTMs include:
- Phosphorylation: This process adds a phosphate group, often acting like a switch to turn the protein's activity on or off.
- Glycosylation: This refers to adding sugar molecules, which can affect how proteins interact with other molecules.
- Methylation and Acetylation: These modifications can alter protein behavior in gene expression and cellular functions.
Post-translational modifications are essential for the regulation of protein functions and the overall cellular control mechanisms.
Understanding folding and modifying proteins provides insight into their functions and how they interact with other molecules within the cell. This knowledge is particularly relevant in fields like biotechnology and medicine, where specific protein functions are necessary for therapeutic purposes.
Researching these processes continues to unveil important aspects of cellular life, enhancing our grasp of essential biological mechanisms.
Regulation of Protein Synthesis
Regulation of protein synthesis is crucial for maintaining cellular homeostasis and adapting to diverse environmental conditions. The process ensures that proteins are produced in the right amounts and at the proper times, which is fundamental for cell function and survival. Different mechanisms control the expression of genes that encode proteins, thus influencing the rates of transcription and translation.
Understanding the regulation mechanisms allows scientists to appreciate how cells react to internal and external stimuli. These mechanisms ensure that proteins are synthesized only when needed, preventing unnecessary energy expenditure and potential production of harmful excess proteins. The implications of such regulation are profound, particularly in the realms of development, metabolism, and responses to disease.
Mechanisms of Gene Expression Control
Gene expression control involves various molecular strategies that impact the synthesis of proteins from genes. This control is multifaceted, incorporating transcription factors, epigenetic modifications, and RNA interference. Each of these components plays a role in determining whether a particular gene is expressed.
- Transcription Factors: These proteins bind to specific DNA sequences and modulate the transcription of genes. They can either promote or inhibit the assembly of the transcription machinery, ultimately influencing RNA synthesis.
- Epigenetic Modifications: Chemical changes to DNA or histone proteins alter gene accessibility without changing the DNA sequence. Examples include DNA methylation and histone acetylation. These modifications can persist through cell divisions, allowing for long-term regulation of gene expression.
- RNA Interference (RNAi): This biological process involves small RNA molecules that can bind to mRNA, leading to its degradation or inhibiting its translation. RNAi thus serves as a critical control mechanism post-transcription, determining how much protein is synthesized.
These complex interactions highlight the gene expression control's intricate nature, allowing cells to precisely tune their protein production to meet their ongoing needs.
Impact of Environmental Factors
Various environmental factors influence protein synthesis regulation. These factors can range from nutrient availability to stress conditions, significantly affecting gene expression.
- Nutrient Availability: The presence or absence of essential nutrients such as amino acids can trigger specific signaling pathways. These pathways adjust protein synthesis rates, ensuring that proteins are produced only when necessary. For example, during amino acid deprivation, cells may degrade existing proteins to recycle amino acids.
- Stress Responses: Environmental stressors, such as heat, oxidative stress, or pathogens, can activate stress response pathways. These responses often result in the synthesis of heat shock proteins and other protective proteins that help the cell cope with adverse conditions.
- Hormonal Signals: Hormones act as messengers that can modulate gene expression during different physiological states. For instance, insulin stimulates protein synthesis in muscle cells, showcasing how hormones directly influence protein production.
> Understanding the impact of environmental factors on gene expression is essential for fields like biotechnology and medicine. By controlling these variables, researchers can optimize conditions for protein production.
In summary, the regulation of protein synthesis is a dynamic process influenced by several mechanisms of gene expression control and environmental factors. Specific adaptations in expression patterns allow cells to respond effectively to changing conditions, which is vital for maintaining life's complexity.
Implications of Protein Synthesis in Biotechnology
The field of biotechnology is profoundly enhanced by the understanding of protein synthesis. The intricate processes of transcription and translation enable the manipulation of genetic material to produce desired proteins. This has far-reaching implications in various sectors, including healthcare, agriculture, and industrial applications. By comprehending how DNA translates to proteins, scientists can devise innovative solutions to complex problems.
One of the primary benefits of this understanding is the capacity for genetic engineering. This involves altering the genetic makeup of organisms to achieve specific traits. For example, organisms like genetically modified crops can have enhanced nutritional profiles or improved resistance to pests. Within the realm of healthcare, protein synthesis plays a critical role in developing vaccines and therapeutic agents.
Applications in Genetic Engineering
Genetic engineering relies heavily on protein synthesis mechanisms, enabling tailored modifications of organisms. Through precise techniques such as CRISPR-Cas9, researchers can edit genes with remarkable accuracy. This results in modifications that can lead to significant advancements in various disciplines, such as medicine and agriculture.
- Disease Resistance: Genetic engineering allows crops to be modified for improved resistance to diseases, reducing the need for chemical pesticides.
- Enhanced Nutrition: By tweaking specific genes, plants can be engineered to contain higher levels of essential nutrients, addressing malnutrition.
- Environmental Adaptation: Modifying the genetic structures in organisms can enable them to thrive in adverse conditions, such as extreme weather or poor soil quality.
Moreover, the ability to introduce or delete specific genes has benefited synthetic biology, which seeks to create novel biological systems. This creates opportunities for innovation, particularly in therapeutics and sustainable biotechnology.
Therapeutic Proteins and Their Development
The development of therapeutic proteins is another crucial implication of understanding protein synthesis. Biopharmaceuticals, such as insulin and monoclonal antibodies, are produced through recombinant DNA technology. This process involves inserting human genes into bacterial or yeast cells, allowing them to produce complex proteins that are otherwise difficult to obtain.
- Insulin Production: Previously extracted from animal sources, insulin is now routinely produced by genetically modified bacteria, ensuring a consistent and ethical supply.
- Monoclonal Antibodies: These proteins are designed for targeted therapies in cancer treatment by binding to specific antigens on cancer cells, marking them for destruction by the immune system.
- Growth Factors: Proteins that stimulate cell growth and development are synthesized for various applications, including treatment of wounds or muscle degeneration.
New advancements in protein synthesis techniques, such as synthetic biology and machine learning, further streamline the development of therapeutic proteins, enhancing efficacy and reducing costs. Research continues to evolve, identifying novel protein structures with potential therapeutic benefits.
"The ability to program living cells offers unprecedented opportunities for medical innovations."
In summary, the implications of understanding protein synthesis in biotechnology are vast and complex. Both applications in genetic engineering and the development of therapeutic proteins stand to benefit tremendously from advancements in this field. The continuing exploration in these areas promises to revolutionize how we approach medical treatment and sustainable practices in the future.
Future Directions in Protein Research
Understanding the future directions in protein research is crucial for enhancing our grasp of molecular biology and its applications. Protein synthesis, being a fundamental biological process, directly affects multiple domains like medicine, agriculture, and sustainability. By investigating these future trends, researchers and practitioners can foster innovation and devise solutions in areas such as disease management, genetic engineering, and environmental protection.
Emerging Technologies
Recent advancements in technology are reshaping the landscape of protein research. Notably, tools like CRISPR-Cas9 have transformed gene editing by allowing precise alterations to DNA sequences. This brings a new level of accuracy and efficiency to genetic modifications. Additional technologies include next-generation sequencing which enhances our understanding of gene expression. Moreover, advancements in mass spectrometry help in analyzing protein structures and interactions at an unprecedented scale. As these technologies evolve, they offer the potential to not only accelerate research but also deliver new therapeutic strategies.
"Emerging technologies are not just tools; they represent a paradigm shift in how we can manipulate biological systems and develop novel applications."
- Integration of high-throughput screening can significantly enhance the discovery of new proteins. This scientific approach allows researchers to analyze thousands of samples simultaneously, increasing the likelihood of finding beneficial proteins.
- The advent of artificial intelligence in protein prediction algorithms promises to streamline the research process by predicting protein structures more efficiently, thereby reducing time and costs associated with experimental trials.
The Quest for Synthetic Biology
The quest for synthetic biology focuses on redesigning organisms for useful purposes by engineering them to have new abilities. Synthetic biology holds immense potential for creating custom proteins, which can lead to breakthroughs in various sectors including healthcare and environmental science. By synthesizing proteins with specific functionalities, researchers can develop smart drugs that respond to individual patient needs or biofuels that are more sustainable than existing options.
Within synthetic biology, significant consideration is given to ethical implications. As we design organisms, we must ensure that these creations do not disrupt natural ecosystems or cause unintended consequences. Furthermore, regulation and public perception will play vital roles in the acceptance of these technologies in society.
As we explore deeper into synthetic biology, understanding the intricacies of protein synthesis remains paramount. The interplay between designing proteins and understanding their natural synthesis pathways is critical. It not only expands our scientific knowledge but also paves the way for innovative applications that can redefine our future.